Quantum LiDAR vs. Quantum Radar
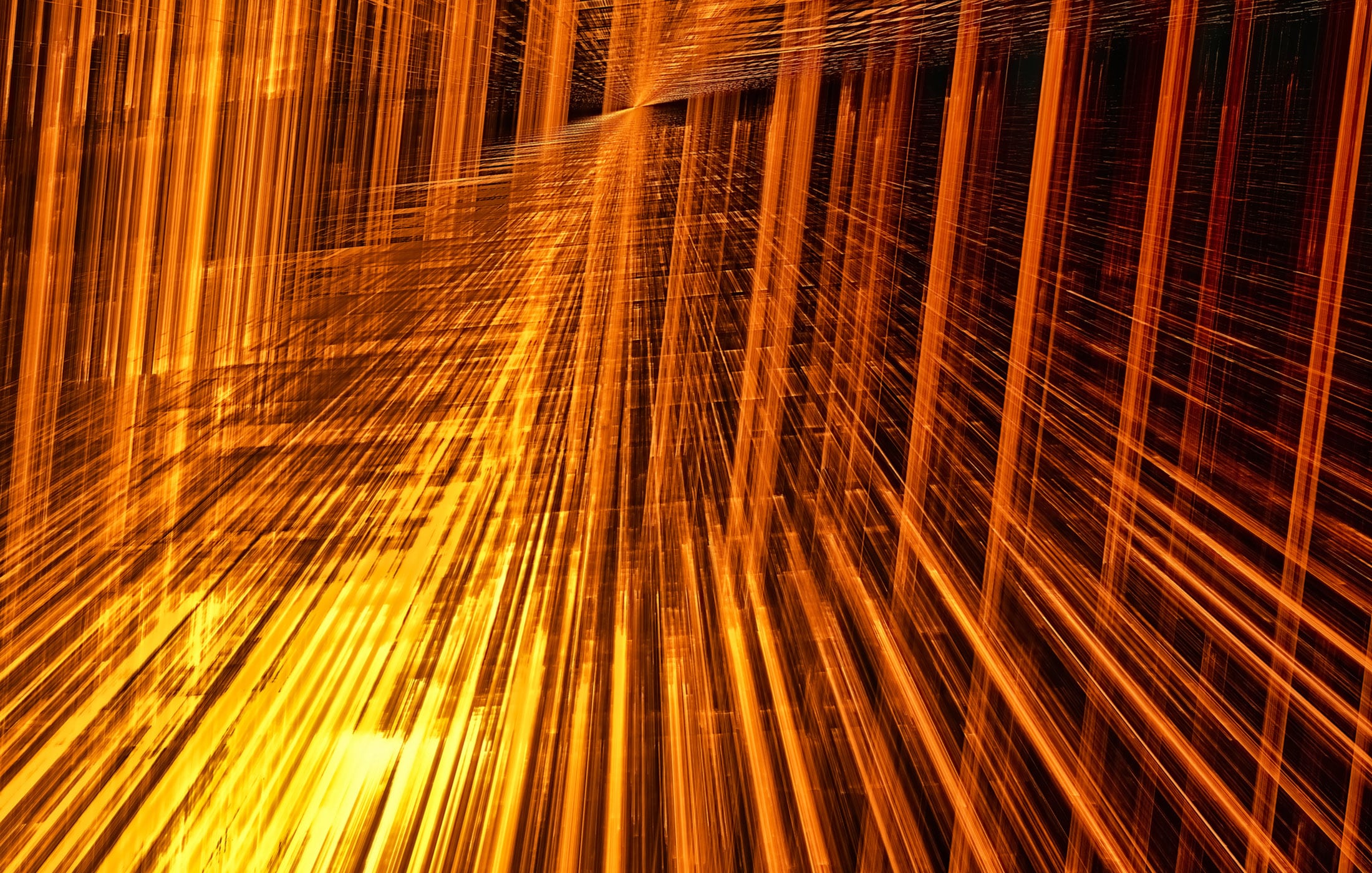
Table of Contents
Introduction
Not long ago, I wrote about the promise of quantum radar, a topic that caught my interest due to its military applications and my defense technology background. Quantum radar has generated buzz for its potential to detect stealth aircraft and resist jamming – naturally drawing attention from defense circles. However, as exciting as it sounds, many readers (and even some tech marketers) have been left confused about how quantum radar differs from quantum LiDAR. Are they the same thing under different names, or fundamentally different technologies? In reality, while both leverage quantum physics to improve detection, they operate on different principles and frequency regimes, leading to distinct strengths and use cases. So let me try and clear up the confusion.
What is Quantum LiDAR?
Imagine a pair of photons (light particles) that are mysteriously connected – a change to one is instantly reflected in the other. This is the spooky phenomenon of quantum entanglement, and it’s at the heart of quantum LiDAR. In a quantum LiDAR system, entangled or other non-classical states of light (like squeezed light) are used to probe the environment. Conceptually, it works a bit like having two linked flashlights: you send one flashlight beam out toward a target (this is the “signal” photon), while you keep its entangled twin (the “idler” photon) at your receiver. When the signal photon hits an object and bounces back, it will be carrying only a very tiny, noisy reflection – so faint it might be impossible to distinguish from background noise using normal methods. But because that returning photon is still quantum-correlated with its twin, the system can compare notes between the twin photons. If the traveling photon encountered something, its “stay-at-home” partner will show corresponding changes. In effect, the entangled partner acts like a witness, helping confirm the presence of a target by matching up with the faint returned signal in a way that random noise cannot. This clever technique is known as quantum illumination – using entangled or correlated light to pull a hidden signal out of noisy surroundings.
Quantum LiDAR, then, is essentially LiDAR enhanced by quantum sensing principles. It still involves sending light out and measuring reflections (just like classical LiDAR), but it uses special quantum states of light to boost performance. By utilizing entangled photon pairs or specially “squeezed” light beams, quantum LiDAR can achieve greater sensitivity and noise resilience than classical LiDAR. In practical terms, a well-designed quantum LiDAR could detect objects with far fewer photons or in far noisier conditions than a conventional system. For example, research demonstrations have shown dramatic improvements in signal-to-noise ratio – in one case, over a 30–40 dB increase in noise tolerance using entangled light, compared to a classical LIDAR approach. This means quantum LiDAR can effectively “see” objects that would be swamped in noise or lost in darkness to a normal sensor. It’s like having night-vision goggles for your LiDAR, allowing it to work in low-light or high-clutter environments where ordinary lasers struggle.
Another powerful aspect of quantum LiDAR is its ability to function at extremely low power levels. Because the detection relies on correlation between photon pairs rather than brute-force reflection intensity, even a weak returning signal can be meaningful. This quantum boost lets quantum LiDAR achieve results with less emitted light energy. In scenarios where it’s beneficial to use as little light as possible (to avoid eye damage, save power, or remain covert), quantum LiDAR has a clear advantage. In fact, the entangled-photon approach inherently adds a layer of security: any interference or eavesdropping on the signal would disturb the delicate quantum state, alerting the system to tampering. This means a quantum LiDAR system could be naturally resilient to spoofing or jamming, an appealing bonus for security and military uses.
To sum up, quantum LiDAR leverages quantum illumination – sending out quantum-linked light and using the spooky coordination between photons to detect what’s out there. By doing so, it offers enhanced precision, better range resolution, improved low-light performance, and strong noise resistance compared to classical LiDAR. Whether peering through a foggy night or scanning a darkened room, a quantum LiDAR can extract meaningful reflections where traditional sensors see only noise. It’s a cutting-edge marriage of photonics and quantum physics that is now moving from theory to experimental reality.
Key Differences Between Quantum Radar and Quantum LiDAR
At a glance, quantum radar and quantum LiDAR sound similar – both use entangled photons to detect objects. But they differ in operating frequency, environmental behavior, and technological maturity. These differences mean each is suited to different scenarios. Let’s break down the key distinctions:
Frequency (Microwave vs Optical): Traditional radar works at radio/microwave frequencies, whereas LiDAR works at optical (infrared or visible light) frequencies. Quantum versions follow the same division. Quantum radar typically uses entangled microwave photons (or microwave signals entangled with optical idler photons), while quantum LiDAR uses entangled optical/near-infrared photons. This fundamental difference in wavelength has huge implications. Microwave photons (used by radar) have wavelengths on the order of centimeters to millimeters, which allows them to penetrate fog, clouds, smoke, and even thin walls or foliage more easily. Optical photons (used by LiDAR) have much smaller wavelengths (hundreds of nanometers), which means higher resolution imaging but also susceptibility to being scattered or absorbed by obscurants like fog or dust. In other words, a quantum radar could see through poor weather or smoke better than a quantum LiDAR, but the quantum LiDAR can see finer detail of what it does detect (like small features or shapes) thanks to its shorter wavelength. This is the same trade-off we see with classical radar vs. LiDAR, now combined with quantum enhancements.
Resolution and Detail vs. Penetration: Because of the wavelength difference, quantum radar and LiDAR excel in different ways. Quantum LiDAR’s optical photons can detect very small objects and map out targets with centimeter or even millimeter precision. It’s like using a fine paintbrush – you can draw a detailed picture of the target. Quantum radar’s microwave photons are more like broad brush strokes – the detail is lower, but you can cover a larger area and even see through certain obstacles. For example, in heavy rain or fog, a quantum radar might still detect an aircraft’s presence when a quantum LiDAR can’t get any return. But if both have a clear line-of-sight to, say, a small drone, the quantum LiDAR could potentially discern the drone’s shape or even identify it, whereas the radar might just register a blob. Operational frequency also means quantum radar could be useful for long-range detection (tens or hundreds of kilometers) in theory, whereas quantum LiDAR will be used at shorter ranges (from a few meters to a few kilometers), similar to how today’s military radars watch the skies while LiDAR scanners map terrain from aircraft or drones.
Environmental Conditions: Building on the above, each technology has different strengths in various environments. Quantum radar (microwave) will perform better in poor visibility conditions – it can penetrate fog, clouds, smoke, or sandstorms much more effectively than optical methods. It might also work at night or day equally (microwaves aren’t affected by ambient light). Quantum LiDAR, on the other hand, might struggle in those same conditions if they are too dense (a thick fog bank could scatter away most photons). However, quantum LiDAR has an ace up its sleeve: noise resilience. Even if there is significant ambient light (like bright sunlight or background radiation that would blind a normal sensor), the quantum LiDAR can pick out the return signal by its quantum correlations. That means in moderate adverse conditions – say light fog, drizzle, or nighttime with lots of background light – quantum LiDAR can still reliably detect objects where a classical LiDAR would be overwhelmed by noise. In summary, quantum radar physically penetrates obstacles better due to its wavelength, whereas quantum LiDAR excels at filtering out noise and interference in the data. Each has an advantage in a different kind of “tough environment”: radar in physically obscured settings, LiDAR in high-clutter or low-signal scenarios.
Power and Stealth: Radar systems (especially military ones) often use high-power radio waves to detect far-away targets. Quantum radar, by contrast, aspires to use entanglement to achieve detection without blasting out massive power – you could operate with much lower energy emissions because you’re gaining sensitivity from quantum effects rather than sheer signal strength. This could make quantum radar harder to detect (a low-power radar is less likely to alert adversaries) and also potentially safer in terms of radiation. Quantum LiDAR similarly can use lower power light pulses than a classical LiDAR for the same reason. A classical LiDAR on a self-driving car, for instance, might use a strong laser pulse that could be eye-hazardous at close range; a quantum LiDAR might achieve equal or better performance with photons spread out in entangled pairs, at much lower intensities. Additionally, both quantum radar and LiDAR offer anti-jamming benefits. In radar, an enemy might try to confuse you with fake signals or overwhelming noise, but a quantum radar can theoretically distinguish genuine entangled return signals from any classical noise or spoofing. The same goes for LiDAR: quantum correlations make it obvious if someone tries to inject a fake echo. This built-in authenticity check is unique to quantum sensing.
Technology Readiness and Complexity: Here lies a major practical difference: quantum LiDAR is currently ahead of quantum radar in experimental progress and ease of implementation. Optical quantum sensing benefits from decades of development in quantum optics (thanks to quantum computing and cryptography research). We have off-the-shelf components like lasers, beamsplitters, and single-photon detectors that operate at or near room temperature. Entangled photon pairs can be generated using relatively compact optical devices (e.g. nonlinear crystals for parametric down-conversion) and detected with sensitive photodiodes. In fact, the first demonstrations of quantum illumination were done with optical photons long before anyone tried it with microwaves. By contrast, quantum radar in the microwave domain is very challenging. Entangling microwave photons or generating squeezed microwave states often requires exotic hardware like superconducting circuits or Josephson parametric amplifiers – and these typically need cryogenic cooling to operate. Handling single microwave photons is tough because thermal noise at room temperature overwhelms them (our environment at 300 K is glowing with microwave noise!). Researchers have managed to demonstrate rudimentary microwave quantum radar prototypes, but they involve lab setups with refrigerators and carefully isolated conditions. One review bluntly noted that microwave quantum radar prototypes so far have extremely limited range – on the order of meters – whereas classical radars easily reach dozens of kilometers. Optical quantum LiDAR, not needing cryogenics, sidesteps one big complexity and thus has been easier to realize in the lab. In short, it’s feasible to build a quantum LiDAR testbed today with available photonic tech, and experiments have already shown its advantages in principle. Quantum radar is catching up, but it’s still a few steps behind in turning theory into a practical device. This is a key reason why many experts expect quantum LiDAR to become useful in real-world applications sooner than quantum radar.
In summary, both quantum radar and quantum LiDAR leverage entangled or quantum-correlated light to beat conventional sensors in certain ways – but quantum radar operates with microwaves for all-weather, long-range coverage, whereas quantum LiDAR uses optical light for high-detail, shorter-range sensing. Quantum radar might be the choice to track a fighter jet through cloud cover, and quantum LiDAR might be the choice to navigate an autonomous car at night or map terrain with extreme precision. They are sibling technologies, each with their own niche.
Use Cases and Market Applications
Because of the differences above, the applications envisioned for quantum radar vs. quantum LiDAR overlap in some areas but diverge in others. Quantum LiDAR, with its likely earlier arrival and finer resolution, is sparking interest in many civilian sectors, while quantum radar remains a hot topic mainly for the military. Let’s explore some key use cases for each:
Military: Both quantum radar and quantum LiDAR have tantalizing defense applications. Quantum radar has been promoted as a “stealth aircraft hunter”, since entangled microwave signals could detect objects designed to evade normal radar. For example, a stealth fighter’s shape and coating scatter regular radar waves away, reducing the echo, but a quantum radar might still pick up a faint signature by correlating returning photons with their entangled twins, effectively pulling the aircraft out of the background noise. Quantum LiDAR could play a similar role at shorter range or in specific scenarios – for instance, tracking stealthy drones or low-flying craft that a ground unit needs to spot. A quantum LiDAR could scan the skies for small drones with a much lower chance of being fooled by decoys or lost in clutter, thanks to its secure photon correlations. Another defense use is high-precision terrain mapping and target identification. Military forces already use LiDAR on aircraft to map the ground (e.g. to generate 3D maps of a battlefield or detect hidden structures). A quantum LiDAR could perform such mapping with fewer photons – potentially allowing a spy drone to map an area covertly, without emitting a easily detectable laser beam. It could also work better at night or through battlefield smoke than current imaging systems. Even submarine detection has been floated as a use: an airborne or satellite quantum LiDAR might detect disturbances or tiny changes in the water surface that hint at a submerged vessel (though this is speculative). In summary, quantum LiDAR could enhance surveillance, targeting, and reconnaissance – complementing quantum radar. Where radar might give the general location of a threat, LiDAR could zoom in and image it in high resolution. Both also offer secure sensing (hard to jam or spoof), which is gold for the military.
Scientific (Space and Earth Observation): The scientific community is excited about quantum LiDAR for applications in space exploration and Earth science. Because quantum LiDAR can work with very low signal levels and still extract a result, it’s ideal for scenarios like planetary mapping from orbit, where only a tiny fraction of light bounces back. Imagine a spacecraft mapping a dark asteroid or the surface of Mars at dusk – a quantum LiDAR could potentially create a 3D map with far fewer photons, saving precious power and possibly seeing details a classical LiDAR would miss due to solar background noise or weak reflections. Similarly, in remote sensing of Earth’s atmosphere, quantum LiDAR could be used to detect trace gases or pollutants by sending entangled photons and filtering out the noisy sunlight background. NASA is already looking into quantum LiDAR for climate and atmospheric studies, with projects aimed at using quantum sensing to improve LIDAR imaging of things like aerosol clouds and greenhouse gas distributions. Because it can handle “photon-starved” environments, quantum LiDAR might, for example, measure greenhouse gas concentrations in daylight conditions that would blind a traditional LIDAR. In astronomy, a quantum LiDAR on a telescope could aid in space debris tracking – picking out small, dark objects in orbit that shine only by reflected light. And there’s even talk of using quantum illumination principles in laser ranging (like how we bounce lasers off the Moon reflectors) to get more precise measurements of distance and perhaps detect beyond the range of classical methods. Overall, the low-signal, high-precision nature of quantum LiDAR is a boon for scientific instruments that need every photon to count.
Commercial & Industrial: This is where quantum LiDAR really stands apart – it has a wide array of potential commercial applications, many of which could be realized in the near to mid-term. A prime example is autonomous vehicles. Self-driving cars today use classical LiDAR sensors to “see” their surroundings, but those sensors can get confused by heavy rain, fog, or certain lighting conditions (like glare). A quantum LiDAR sensor in a future autonomous car could retain much better performance in these challenging conditions. It could, for instance, detect another car through road spray or a pedestrian on a dark, foggy night – scenarios where current sensors might fail. Enhanced precision and range from quantum entanglement could also give the car more notice of obstacles down the road. Companies in the automotive industry are keenly watching quantum sensing developments, since any improvement in reliability or safety is a huge win for self-driving tech. Beyond vehicles, industrial automation and robotics could use quantum LiDAR for tasks like high-precision 3D scanning and navigation in complex environments. Picture a robotic forklift in a warehouse full of dust or smoke – a quantum LiDAR-based vision system could safely guide it where a normal one might be blinded. There are also possibilities in medical imaging: using LiDAR-like depth sensing for surgeries or diagnostics, where quantum illumination might enable imaging of tissue with very low light (to avoid damage) or through optically thick media. For example, a quantum LiDAR could form the basis of a next-gen endoscope or imaging device that works with minimal light inside the body, relying on the correlations of photons to form an image even amidst scattering. In disaster response, firefighters could deploy a quantum LiDAR drone to scan a smoke-filled building for survivors – the drone’s entangled-photon sensor might pick up the faint outline of a person through smoke and darkness, succeeding where a thermal camera or regular LIDAR gets overwhelmed by interference. The security industry might also benefit: think of perimeter sensors or scanning devices that need to spot intruders or weapons with high certainty. A quantum LiDAR scanner at an airport, for instance, could potentially detect a hidden object by seeing a subtle shape under someone’s clothing (using safe levels of light) and not be fooled by intentional jamming devices. While these commercial uses are still on the horizon, they illustrate how broadly applicable quantum LiDAR could be outside of the military – far more so than quantum radar, which isn’t very practical for, say, your Tesla or a firefighter’s toolkit.
Niche Applications: Finally, there are some niche but fascinating areas where quantum LiDAR might shine. Underwater mapping is one: Lasers don’t travel far in water, but they are used in coastal mapping (bathymetry) and undersea lidar for short ranges. A quantum LiDAR could potentially see a bit further or get clearer images underwater by squeezing out more information from the few photons that aren’t scattered. In fact, a quantum LiDAR prototype was recently shown to capture 3D images underwater with very high resolution, demonstrating the feasibility of quantum sensing in sub-sea environments. High-resolution archaeology is another niche – archaeologists use airborne LiDAR to uncover ruins hidden under forest canopies or to scan historical sites. Quantum LiDAR could improve the resolution of these scans, or require fewer passes to get quality data, which is helpful when trying to map delicate or hard-to-reach sites without disturbing them. It might even enable “quantum ground penetrating LiDAR” by picking up tiny differences in returns that could indicate buried structures. Similarly, structural inspection (like detecting minute cracks in infrastructure) could benefit: a quantum LiDAR could possibly detect smaller flaws at longer distances than current optical scanners, by virtue of improved signal processing. And in the realm of communications, while not exactly LiDAR, the same quantum LiDAR hardware might double as a quantum communications device (since entangled photons can be used for ultra-secure communication when not used for sensing). This points toward hybrid systems in the future that do both sensing and communication with quantum states of light.
Who is Leading the Research and Development?
The race to develop quantum radar and LiDAR is truly global, with a mix of government, academic, and industry players pushing the frontiers. Here’s an overview of who’s at the helm of this cutting-edge R&D:
Government & Defense Initiatives: Given quantum radar’s military appeal, it’s no surprise that defense agencies are heavily funding research. In China, the National Quantum Laboratory and defense conglomerates like CETC (China Electronics Technology Group Corporation) have claimed major strides. Back in 2018, CETC announced a prototype quantum radar purportedly capable of tracking high-altitude objects and detecting stealth aircraft. While details are scarce (and some skepticism is warranted), China’s publicized efforts suggest a serious government-backed push in quantum radar tech. Canada has also invested in quantum radar: Canada’s Department of National Defence funded a project at the University of Waterloo to build a quantum radar demonstrator aimed at detecting stealth targets. This brought Canadian industry (notably Lockheed Martin Canada as a partner) into the game alongside academic researchers. In the United States, DARPA (Defense Advanced Research Projects Agency) has launched programs related to quantum sensing – for example, the Quantum Apertures program focuses on new ways to receive radio-frequency signals using quantum effects, which is closely related to radar capabilities. DARPA’s Robust Quantum Sensors (RoQS) initiative likewise aims to take quantum sensors out of the lab and make them field-ready, which could include quantum radar/LiDAR systems for platforms like aircraft or satellites. The EU is not left behind either. The European Union’s Quantum Flagship program, a €1 billion initiative, has a dedicated segment for quantum metrology and sensing. European researchers are working on quantum-enhanced LiDAR under this umbrella, often targeting applications like automotive sensors or imaging. The UK, via its national Quantum Technology Hubs, has a program called QuantIC (Quantum Imaging Hub) which explicitly works on quantum LiDAR and radar concepts (in partnership with companies like BAE Systems). In fact, BAE Systems has shown interest in quantum sensing for future defense systems, and was involved in projects to detect small drones using quantum-enhanced sensors. All told, many governments have recognized that quantum sensors could be strategic game-changers, and they’re funding national labs, universities, and defense contractors to lead the R&D charge.
Academic Research Labs: A lot of the foundational work for quantum radar/LiDAR comes from university labs and institutes. MIT, for example, produced seminal proposals for quantum illumination (Seth Lloyd’s work in 2008) and researchers like Jeffrey Shapiro have experimentally explored quantum LiDAR concepts. The University of Toronto and the National Institute of Standards and Technology (NIST) collaborated on a quantum radar experiment in 2019 that successfully detected a target at short range using microwaves entangled with an optical idler, a noteworthy milestone. The Institute for Quantum Computing at University of Waterloo in Canada (mentioned above) has been a leader, demonstrating a prototype quantum radar using two-mode squeezed microwave states. In Europe, groups in Italy and the UK have been actively publishing quantum LiDAR studies – for instance, researchers at the University of York and University of Sheffield (UK) have authored reviews on quantum radar and performed experiments on quantum illumination in optical domains. Austrian and German labs have looked at quantum-enhanced laser ranging and Doppler LiDAR. There’s also significant work in Australia and Singapore on quantum LIDAR prototypes using photonic chip technology. Many of these academic efforts are now showing up in high-profile journal papers and conferences, indicating that the science is rapidly progressing.
Private Sector & Industry: On the commercial side, certain companies and startups are staking claims in quantum LiDAR, sensing an opportunity to create the next generation of sensors. One notable example is Quantum Computing Inc (QCI), a U.S.-based company that, despite its name, is also heavily into quantum sensing. QCI has developed a quantum LiDAR prototype and even sold one to an end-customer for testing – a prototype capable of underwater 3D imaging (for mapping undersea environments). They have also secured multiple contracts with NASA to advance quantum LiDAR for Earth observation and climate research, showing that there is commercial interest in deploying quantum LiDAR in real-world applications (like airborne or satellite remote sensing). Another startup, Aegiq (in the UK), and QuiX (in the Netherlands) are developing quantum photonic technologies that could be applied to LiDAR and imaging. Big tech companies in the photonics and defense space are also circling. For example, Honeywell and Lockheed Martin have quantum research divisions that, among other things, look at quantum sensors for navigation and imaging. BAE Systems (UK/U.S.) and Raytheon have mentioned quantum sensing in their future technology roadmaps. It’s telling that even automotive LIDAR companies (like those making sensors for self-driving cars) are keeping an eye on quantum—though they aren’t selling quantum LIDAR yet, some have partnerships with research institutions to be ready to integrate quantum tech when it matures. Finally, we should mention that the aerospace industry (e.g., companies like Airbus or Thales) are interested in quantum LiDAR for aviation safety and space situational awareness. In short, R&D leadership is a mix: governments fund the hard stuff, academics prove it can work, and companies aim to be the first to market with a product, whether that’s a quantum-enhanced sensor for a car or a quantum radar for a fighter jet.
Challenges and Feasibility of Deployment
With all the excitement around quantum radar and LiDAR, it’s important to temper expectations with a look at the challenges. Both technologies face significant hurdles before they can be widely deployed outside the lab. Let’s examine the key issues and what they mean for real-world feasibility:
Range and Signal Loss: Perhaps the most glaring challenge is effective range. Entanglement and quantum states are delicate – they don’t survive long when sent through a lossy medium. This means the distance over which a quantum radar or LiDAR can reliably operate is currently quite limited. Analyses of microwave quantum radar suggest that for now the maximum range is on the order of a few tens of meters for detecting something like an aircraft, far shorter than classical radars. Optical quantum LiDAR can also suffer from loss over distance (air absorbs and scatters light). While you might not need entanglement to survive all the way to the target and back – quantum illumination can work even if entanglement is mostly broken, as long as some correlation remains – the fact is that distance plus noise dilute the quantum advantage. Over long distances, you eventually collect so little signal that even quantum tricks can’t help. Researchers are exploring ways to extend range: for instance, using quantum repeaters or transducers that convert entangled optical photons to microwaves (so you get the best of both worlds: low-loss microwave propagation with easy optical entanglement generation). Still, for the near future, we expect quantum LiDAR to be used in relatively short-range scenarios (within a few kilometers at best, more likely sub-kilometer for high resolution) and quantum radar to remain a short-range demonstrator until breakthroughs occur.
Cryogenics and Complexity: As mentioned earlier, quantum radar often requires cryogenic equipment. Superconducting single-photon detectors and microwave amplifiers may need temperatures just a few degrees above absolute zero to function with low noise. This is obviously a deployment nightmare – you can’t easily put a fridge-cooled quantum radar on a fighter jet or a satellite in its current form. Quantum LiDAR has an easier path here: many quantum LiDAR setups can use solid-state SPAD detectors (single-photon avalanche diodes) or emerging photonic detectors that operate at cooler temperatures (perhaps requiring only Peltier cooling or none at all). Additionally, aligning and maintaining entanglement or squeezed states requires stable optics and precise timing. Phase stability is critical; any phase noise can wash out the quantum correlations. In a controlled lab, you can stabilize things on an optical table, but out in the field, vibrations, temperature changes, and platform motion (like an airplane moving) could disrupt the delicate quantum states. Researchers are working on making quantum LiDAR more robust – for example, using phase-insensitive receivers that don’t require perfect phase alignment. But until these systems are hardened, deploying them will be tricky.
Photon Source and Detector Limitations: Generating a stream of entangled or single-photon states with high brightness is non-trivial. Most entangled photon sources (like SPDC crystal setups) only produce a tiny fraction of pairs per laser pulse. If you try to increase the rate, you often introduce noise or lose the quantum properties. Likewise, single-photon detectors can be saturated or overwhelmed by too much light, yet they also have finite efficiency (not every photon is detected) and some false-count rate (dark counts). Current quantum LiDAR prototypes often have to balance these trade-offs, using short-range targets and long acquisition times. As one summary put it, today’s single-photon sources suffer from “low brightness and limited photon generation rates,” which hurts long-range performance. Improving this might require new tech like quantum dot photon sources or more efficient nonlinear materials, or multiplexing many entangled sources in parallel to boost the rate. On the detector side, there are advances like superconducting nanowire detectors (SNSPDs) that have very high efficiency and low noise, but again they need cryogenic cooling and are expensive. The technology is steadily improving – for instance, integrated photonic chips might one day generate entangled photons on-chip and direct them to built-in detectors, greatly reducing the system’s footprint and complexity. Until then, scaling a quantum LiDAR to have both long range and fast update rate (for real-time scanning) remains a challenge.
Computational and Data Processing Needs: A perhaps under-appreciated challenge is the data processing in quantum sensing. In a classical radar or LiDAR, you send out a pulse and you get back echoes that you can directly time and map. In a quantum system, you are often dealing with correlation measurements – essentially looking for a tiny statistical signature of a target in a huge sea of noise. This can demand heavy computational filtering. You might need to store and compare large streams of photon detection events between the signal and idler paths to find matches. Advanced algorithms (potentially quantum algorithms, or classical machine learning) could be needed to sift the data quickly. There’s progress here: some experiments have used fast coincidence counting electronics or even real-time quantum signal processing to demonstrate quantum advantage in detection. But if, say, an autonomous vehicle were to use quantum LiDAR, it would need a processor capable of handling the quantum sensor’s output on the fly. This adds to the development complexity. The good news is that classical computing power is enormous today and can likely handle these tasks for moderate sensor rates; still, optimizing the system so it’s not only quantum-optically efficient but also computationally efficient will be key to practical use.
Cost and Integration: As of now, quantum radar and LiDAR setups are expensive research prototypes. They involve specialized lasers, nonlinear crystals, single-photon detectors, perhaps vacuum or cryogenic systems, and precise timing electronics. Early deployment will likely happen only where the performance boost is so crucial that the cost is justified (e.g., military systems or very high-end scientific instruments). Over time, just as GPS or infrared cameras eventually shrank and became affordable, we’d need quantum sensing components to go down the cost curve. This typically happens with volume production and tech maturation – possibly driven by the photonic integrated circuit industry. Another practical concern is size/weight: a quantum LiDAR on a satellite must be compact and lightweight, and an array of quantum radar sensors on an aircraft should ideally fit the existing form factor. Miniaturization is a challenge but not an impossible one – there’s active research into integrating sources and detectors on chips, as mentioned, and even into solid-state quantum LiDAR (tiny devices that could be the quantum analog of today’s MEMS LiDAR units). Regulatory issues might also arise: for quantum LiDAR, using entangled photons doesn’t pose new safety issues beyond what standard lasers do (eye safety standards still apply), but using certain wavelengths might require spectrum clearance if it interferes with other systems. Quantum radar, operating at RF, might need to use frequency bands carefully to not conflict with communications – though one advantage is it could possibly operate with such low power (below noise floor) that it’s effectively undetectable to others.
Given these challenges, which technology is likely to see real deployment first? Most experts bet on quantum LiDAR leading the way. The relative maturity of optical quantum hardware and the immediate commercial interest in better LiDAR (for cars, drones, etc.) mean that we’ll probably see quantum-enhanced LiDAR units in the field within the next several years. They might appear as specialized sensors for niche markets at first – for example, a quantum LiDAR for high-end drones that need to navigate in low-visibility conditions, or for a research aircraft mapping atmospheric gases. Over time, as costs come down, they could trickle into more common applications like autonomous vehicles or industrial sensors. Quantum radar, on the other hand, will likely stay in the defense realm a while longer. We might see a demonstration of a short-range quantum radar on a naval ship or as a ground-based sensor to detect stealth aircraft or incoming missiles, but widespread use (like mounting on every fighter jet or air-defense system) is further out. It’s also possible we’ll first see hybrid approaches – for instance, classical radar systems augmented with quantum receivers for better sensitivity (a sort of halfway quantum radar), or classical LiDAR that incorporate a quantum channel for higher fidelity in certain modes. Such incremental adoption could be a stepping stone before fully quantum systems are common.
Conclusion and Future Outlook
Quantum radar and quantum LiDAR represent a new frontier in sensing, bringing the strange advantages of quantum mechanics to the macroscopic world of detecting planes, cars, and landscapes. We’ve seen that despite sharing some concepts, the two are distinct in their operational domains: quantum radar uses entangled radio/microwave waves, aiming to excel in long-range and all-weather detection (with stealth-defying capabilities), while quantum LiDAR uses entangled light beams, offering high-resolution, noise-tolerant sensing that is likely to find use in many civilian technologies first. The confusion in the market about these terms is understandable – both promise “quantum-enhanced detection” – but as we’ve clarified, their differences in frequency and use cases mean they will likely complement, not replace, each other. Quantum LiDAR is the one to watch for near-term impacts in everyday life, from safer autonomous navigation to scientific breakthroughs in environmental monitoring. Its relative technical maturity and broad applicability give it a head start outside the military domain.
Looking ahead, the future of quantum sensing is bright – and not just limited to radar and LiDAR. The next decade could see quantum versions of other sensors (like quantum magnetic sensors, quantum gravitometers, etc.) merging into a new ecosystem of ultra-sensitive instruments. For radar and LiDAR specifically, we can expect steady improvements: increased range, smaller hardware, and integration with classical systems. One can imagine a future stealth aircraft that is still detectable, not by a giant radar dish, but by a network of quantum radar satellites quietly picking up its trail through entangled microwave signals. Conversely, one can imagine a fleet of autonomous taxis driving with virtually flawless vision, because each has a quantum LiDAR that never gets blinded by sun or confused by fog. There’s also exciting potential in hybrid sensing solutions – for instance, a system that uses both quantum radar and quantum LiDAR in tandem. A drone could have a microwave quantum sensor to probe through foliage and an optical quantum LiDAR for fine imaging once an object is found. These combined approaches could yield an unprecedented level of awareness, basically a quantum multi-spectral vision.
We should also consider how quantum sensing might evolve with quantum computing and communications. Some visions of the future see quantum sensor networks, where sensors are entangled with each other or with quantum computers to instantly share information or enhance sensitivity over large baselines. It’s conceivable that a quantum radar on one coast and a quantum radar on another could be linked via entanglement to jointly detect a distant object in a way that boosts confidence and reduces false alarms (very speculative, but theoretically not impossible). Quantum LiDAR might integrate with quantum communication links to securely send its findings to a cloud, all encrypted by the laws of physics.
In conclusion, quantum radar and quantum LiDAR are no longer science fiction – they are emerging reality, albeit in early stages. They differ in technology and likely timelines: expect to hear more about quantum LiDAR in commercial products soon, while quantum radar will continue to be a strategic project for defense and require further breakthroughs to reach its full promise. Both, however, underscore the transformative power of quantum technology. As these sensors evolve, they could redefine how we perceive the world, achieving what was once thought impossible – like spotting the “invisible” stealth plane, or navigating a pitch-black, foggy road with the same confidence as a clear day. The coming years will be critical in turning lab experiments into robust devices. If successful, the result will be a new class of quantum-enhanced eyes on our world, sensing further, clearer, and more securely than ever before.