Quantum Sensing – Introduction and Taxonomy
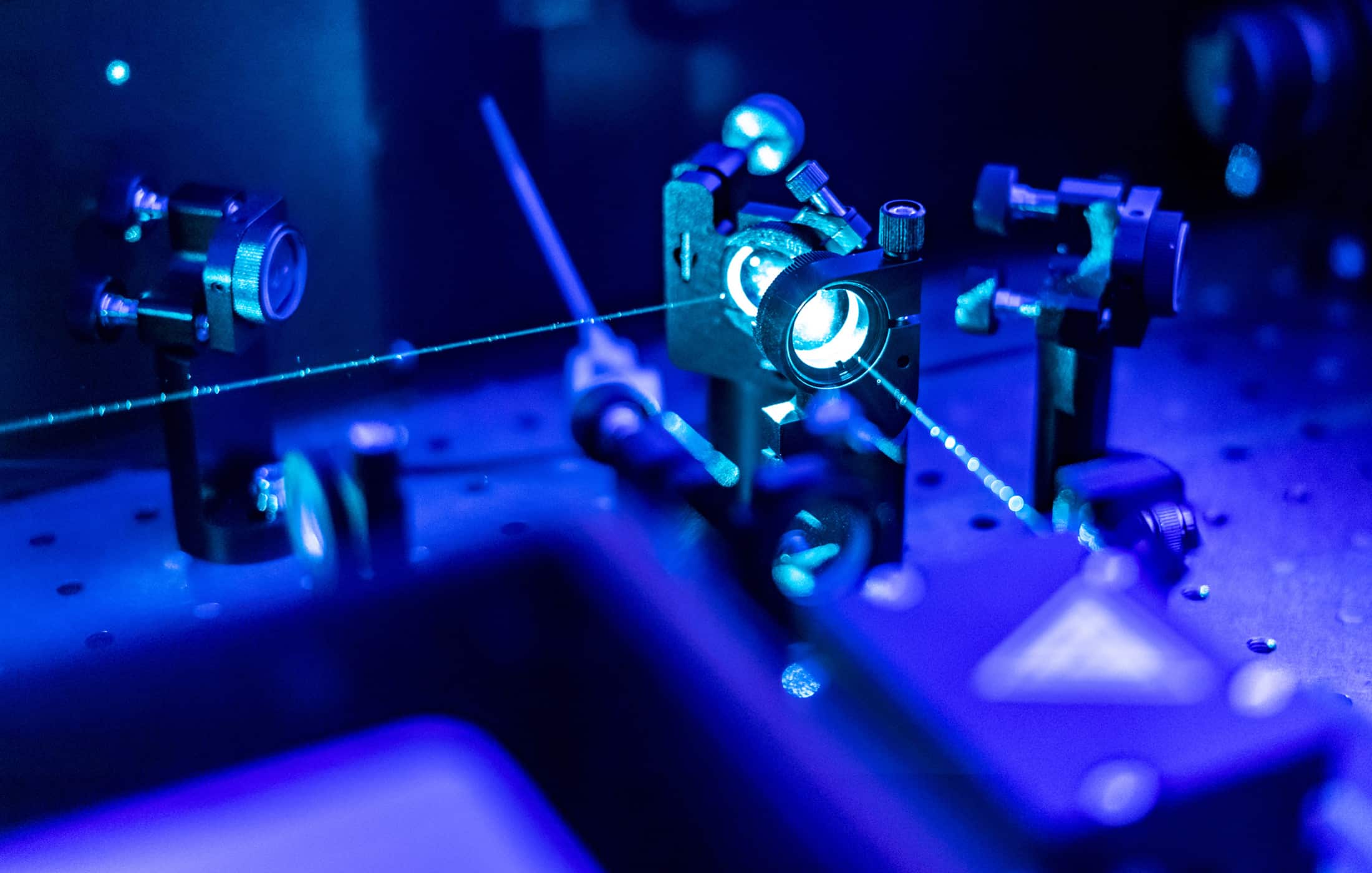
Table of Contents
Introduction
Quantum sensing—the science of exploiting quantum phenomena like entanglement, superposition, and squeezed states—represents a dramatic leap in how we measure and interpret the world around us. While many modern instruments already rely on quantum principles (e.g., lasers in optical scanners, quantum transitions in atomic clocks), the new wave of quantum sensors takes this integration further. By intentionally harnessing quantum effects to surpass classical limits, these devices promise sensitivity and precision that could revolutionize industries from healthcare to defense.
Much of the early theoretical groundwork for quantum sensing was laid in research by Lloyd (2008) on quantum illumination, and later expanded by Tan, Pirandola, and Shapiro (2009), demonstrating how entangled photons might improve target detection in noisy environments. That same decade saw accelerating progress in cold-atom sensors (Kasevich & Chu, 1991; Peters et al., 1999), which proved matter-wave interferometry could yield ultrahigh sensitivity for measuring gravitational fields. Seminal developments like these inspired further exploration of specialized sensing platforms, from superconducting quantum interference devices (SQUIDs) in magnetometry to nitrogen-vacancy (NV) centers in diamond for magnetic, thermal, and electric field sensing.
Despite the rapid advancements, key challenges remain before quantum sensors achieve widespread deployment. Fragility and environmental isolation requirements often restrict these sensors to laboratory settings. Efforts in miniaturization and packaging—such as chip-scale atomic clocks and vapor-cell magnetometers—are bridging the gap, but cryogenics, specialized laser systems, and the need for robust shielding continue to limit practical rollout. Moreover, standardization and calibration pose significant hurdles. Many quantum devices are assembled in small batches without established guidelines for comparing performance, creating an additional barrier to mass adoption and regulatory oversight. Yet as R&D groups refine manufacturing techniques, develop noise-robust designs, and cultivate supply chains for specialized materials, quantum sensors edge closer to real-world viability.
Underpinning this field is a growing sense of taxonomy—the recognition that “quantum sensing” is not monolithic but an umbrella term covering diverse technologies. From atom/ion-based sensors (e.g., atomic clocks, inertial sensors, gravimeters) to photonics and light-based devices (quantum LIDAR, ghost imaging, quantum radar), magnetometry (SQUIDs, NV centers, alkali vapor cells), RF/microwave sensors (Rydberg-atom receivers, superconducting resonators), and niche systems like quantum thermometers or optomechanical sensors—each category employs unique quantum phenomena and engineering strategies. Together, they shape a landscape where breakthroughs in one arena often spark improvements in another, illustrating the synergy that characterizes the quantum revolution.
Ultimately, the societal impact of these sensors could be enormous. In healthcare, quantum magnetometers might enable portable brain-imaging devices, revolutionizing neurology and mental health diagnostics. In defense, quantum inertial sensors promise GPS-free navigation and a robust way to detect submarines in the ocean depths. For basic science, hypersensitive atomic clocks and quantum gravimeters offer deeper insight into everything from Earth’s changing climate to gravitational-wave detection. Although the journey from laboratory prototypes to ubiquitous commercial devices is not trivial, the trajectory is clear: as research and engineering mature, quantum sensing will likely unfold new frontiers—pushing both our technological capabilities and scientific understanding far beyond what was once thought possible. For more on quantum sensing use cases, see: Quantum Sensing: Reimagining How We Perceive Reality – Key Use Cases.
Theoretical Groundwork
Each of the papers below and researchers has contributed pieces of a puzzle that is now coming together. The field’s literature is rich, from foundational theory in the 80s and 90s, to experimental breakthroughs in the 2000s and 2010s, to modern demonstrations crossing into engineering. The collective progress built a toolkit of quantum techniques – entanglement, squeezing, single-spin control, atom trapping, etc. – that is enabling today’s quantum sensors.
Ghost Imaging Demonstration (Shih et al., 1995): In a remarkable experiment, physicists produced an image of an object using entangled photons where one photon of each pair never went near the object. This “ghost imaging” concept showed how quantum correlations can be used to form images with fewer particles or through noisy media. It was initially a quantum optics curiosity, but has since inspired advanced imaging techniques (quantum LiDAR and biomedical imaging through scattering tissue) using both quantum and classical correlations. The 1995 demonstration by Pittman, Shih, and colleagues is considered a milestone in quantum imaging.
Quantum Illumination & Entanglement in Radar (Lloyd & Shapiro, 2008–2009): Seth Lloyd’s 2008 paper on Quantum Illumination and a 2009 follow-up by Tan, Pirandola, Shapiro developed the theory that entangled light could improve target detection in noisy environments. They showed a theoretical 6 dB (factor of 4) improvement in error probability by using entangled signal-idler pairs over the best classical strategy. This work is the basis of quantum radar concepts. While a full-scale quantum radar hasn’t yet been realized, these papers are seminal in applying entanglement to a practical sensing protocol. The media hype around “quantum radar” often traces back to these theoretical papers.
Precision Gravimetry with Cold Atoms (Peters, Chung, Chu 1999; Kasevich & Chu 1991): Steven Chu’s group pioneered atom interferometry for gravity sensing. By 1999, Mark Kasevich (Chu’s former student) and colleagues demonstrated an atom interferometer gravimeter with $$10^{-9}g$$ sensitivity. Their techniques are the foundation for today’s quantum gravimeters. Recently, the Birmingham group’s Nature paper (2022) reported the first outdoor use of a quantum gravity gradiometer to detect a tunnel, a breakthrough led by Michael Holynski and Kai Bongs. In that paper, Bongs famously stated it was an “Edison moment in sensing,” underlining its transformative importance.
Optical Frequency Combs (Hall & Hänsch, 2005 Nobel; Ye et al.): The frequency comb isn’t a sensor by itself, but it enables many quantum sensors (optical clocks, precision spectroscopy). Theodore Hänsch and John Hall’s development of the comb in the late 1990s (Nobel Prize in 2005) revolutionized metrology by allowing optical frequencies to be counted. Jun Ye’s group integrated combs with optical lattice clocks, leading to the 2017 record clock comparisons. A 2017 Science paper by Ye’s team compared two clocks at the $$10^{-18}$$ level by linking them with a comb, effectively using one quantum sensor to calibrate another.
Entangled Clock Networks (Komar et al., 2014): A theoretical proposal by Peter Komar and colleagues showed that networks of entangled clocks could measure time differences (or gravitational potential differences) with better precision than separate clocks. This idea has influenced ongoing research in linking optical clocks and even using them to detect gravitational waves or dark matter. It’s a forward-looking example of how quantum sensing and quantum networks might merge.
Notable Researchers: Many individuals have driven quantum sensing forward. To name a few: John Kitching (NIST) for chip-scale atomic magnetometers and clocks (his 2005 work on a microfabricated Cs clock made “quantum sensors on a chip” a reality); Alexander Pines and Dmitry Budker for applying atomic magnetometers to NMR and exotic physics searches; Jian-Wei Pan (USTC, China) who, beyond quantum communication, has advanced quantum precision measurement in space (quantum experiments on satellites); Vladan Vuletić (MIT) and Monika Schleier-Smith (Stanford) for experiments on spin-squeezing in large atom ensembles, pushing quantum sensor performance; and many others.
(For readers seeking more depth, the 2017 Reviews of Modern Physics article “Quantum Sensing” by Degen, Reinhard, and Cappellaro is an excellent overview, and the 2020 roadmap “Quantum Metrology and Sensing” in Journal of Applied Physics provides a comprehensive snapshot of the field and key references.)
Key Challenges and Roadblocks
With all the promise of quantum sensing, it’s equally important to discuss the challenges and roadblocks that must be overcome before these technologies reach their full potential. Quantum sensors face technical hurdles, commercial and scalability issues, and even regulatory and adoption challenges. Let’s examine some of the most significant:
Fragility and Environmental Isolation
Quantum systems are famously delicate. Many quantum sensors require carefully controlled environments – vacuum chambers for cold atoms, cryogenic temperatures for superconducting devices like SQUIDs, or heavy magnetic shielding for sensitive magnetometers. Outside a lab, maintaining these conditions is hard. Vibration, temperature fluctuations, stray electromagnetic fields – all can disrupt a quantum sensor’s operation or introduce noise. For example, an atomic interferometer on a moving vehicle needs vibration isolation so it can tell apart a gravity signal from a jolt in the road. Likewise, an optically pumped magnetometer in a hospital must deal with magnetic noise from elevators, cars, etc.
While techniques exist (common-mode noise cancellation, feedforward stabilization), making quantum sensors robust to real-world noise is a non-trivial engineering challenge. The extreme sensitivity that is their strength is also a weakness: without careful filtering, they pick up everything. As the Boston Consulting Group (BCG) noted, the “extreme sensitivity of quantum sensors” is both their greatest strength and greatest weakness, since uncontrolled noise can corrupt results outside the lab. Overcoming this means better shielding, smarter data processing, and feedback systems to stabilize sensors.
Size, Weight, and Power (SWaP)
Many quantum sensors today are too bulky or power-hungry. A lab optical clock might fill a table and consume kilowatts of power for lasers and vacuum pumps. A quantum gravimeter can be the size of a refrigerator. For widespread use, these need to shrink and become more energy-efficient. There is progress – e.g., NIST’s chip-scale atomic clock is under 100 cm³ and uses just 100 mW. But going from one specialized device (like a clock) to complex sensors (like an entangled magnetometer network) requires integrating lasers, detectors, and sometimes cryogenics into a compact package.
Miniaturization is underway via microfabrication of vacuum cells, photonic integrated circuits for guiding laser light, and even on-chip cooling techniques. Still, reaching a point where (for instance) a quantum gyro can fit on a circuit board next to a MEMS chip will take considerable R&D. Size and power constraints also link to environments: a submarine might accommodate a large quantum inertial sensor module, but a car or smartphone cannot. So different applications have different SWaP targets. Achieving the extreme performance in a small form factor is perhaps the defining engineering battle for quantum sensing in the 2020s.
Complexity and Cost
Presently, quantum sensors are often handcrafted devices operated by PhDs. The systems integration – lasers, optics, electronics, firmware – is complex. This complexity drives up cost and lowers reliability. A commercial sensor needs to be operable by a technician, not a quantum physicist. That demands automation and simplification of operation (e.g., automated laser locking, self-calibration routines). Cost is a huge factor: if a quantum gravimeter costs $500k, only a few geophysics companies might buy one; if it costs $50k, it becomes far more accessible. Cost should come down with volume manufacturing and as designs mature (today’s devices are often prototypes built in low numbers). Government investments are helping push units toward manufacturability – for instance, quantum tech hubs working with industry to create supply chains for components (like specialized laser systems, vacuum packages) so that each new sensor needn’t reinvent the wheel.
Still, it’s a catch-22: costs won’t drop until volume increases, but volume awaits a compelling reason (performance advantage) to adopt the tech. This is why initial markets may be niche (defense, etc.) where cost is less sensitive; as those early adopters fund development, costs can drop for broader markets.
Comparison with Improving Classical Sensors
A subtler challenge is that classical sensor technology is not standing still. MEMS inertial sensors, for example, keep improving in precision; classical LIDAR is advancing rapidly; new algorithms improve classical radar resolution and sensitivity. Quantum sensors must offer significant improvements or unique capabilities to justify a switch. In many cases, classical and quantum will coexist, each used where it’s best suited.
There is a risk that for some applications, by the time quantum sensors are deployable, classical tech might have narrowed the gap (through clever engineering, sensor fusion, AI-based signal processing, etc.). Therefore, quantum projects often target what classical absolutely cannot do (like detecting DC gravity signals, or sensing single molecules non-invasively).
It’s important for quantum sensor developers to keep an eye on competing technologies to ensure they aim for genuine breakthroughs, not just incremental gains that could be achieved classically at lower cost. The BCG report pointed out that in some industries, unclear performance advantage combined with high upfront costs makes adoption unattractive. Clear advantage must be demonstrated.
Scalability and Production Challenges
Scaling up production of quantum sensors is non-trivial. Many devices rely on components that are painstakingly tuned by experts. For instance, fabricating diamond with perfect NV centers or ultra-low-noise lasers for atomic sensors still verges on art. To mass produce, you need quality control and standardization. Right now, lack of standardization is a barrier – every lab uses slightly different techniques. As an analogy, think of early transistors in the 1950s, each one handmade vs. modern semiconductor fabs churning out billions. Quantum sensing might need its version of the “fab”. Companies will have to invest in manufacturing processes, possibly borrowing from photonics and semiconductor industries, to make hundreds or thousands of identical quantum sensors. Until then, each unit may be slightly bespoke, affecting unit-to-unit reliability and calibration.
Also, exotic materials (superconductors, isotopically pure diamonds, rare isotopes of gases) might face supply limitations or high costs. Ensuring supply chains for these – and doing so at scale – is part of scalability. On scaling up the sensor size (like arrays of sensors or larger baseline interferometers), challenges include maintaining entanglement or phase stability over large distances. Distributing entanglement for sensors in different locations (a network of atomic clocks or magnetometers) requires quantum networking techniques that are still being refined.
Cryogenics and Cooling Requirements
Some of the most sensitive sensors (SQUIDs, certain optomechanical sensors, superconducting resonators for THz detection) need millikelvin temperatures, provided by dilution refrigerators or liquid helium. This is a huge operational hurdle – cryogenics is power-intensive and not portable except in niche cases. There’s a push to develop high-$$T_c$$ superconducting sensors (to raise operating temp) or to find room-temperature alternatives (like NV centers replacing SQUIDs in some use cases ). Until then, where cryogenics are unavoidable, that confines those sensors to lab or fixed installations and increases cost.
The necessity of lasers is another parallel – less extreme than cryogenics, but high-performance lasers are power-hungry and need stabilizing. However, laser technology has improved such that compact, rugged lasers (telecom diode lasers, fiber lasers) can sometimes be used instead of big research lasers. It’s a challenge being actively addressed: creating “turn-key” lasers for quantum sensors, akin to how laser companies now sell pre-stabilized units to quantum labs.
Calibration and Standardization
Users will want to trust the readings of a quantum sensor. This requires calibration against known standards. Traditional sensors have decades of calibration procedures established. For some quantum sensors, new methods are needed. For example, how do you calibrate a quantum gravimeter for absolute accuracy? One must compare it with a known reference (perhaps a conventional gravimeter or dropping a weight and timing it). There might be systematic biases unknown in new devices.
As quantum sensors get commercialized, organizations like NIST or NPL will likely create calibration services and reference standards. Additionally, standards for performance reporting need to be set (so that different makers can be compared apples-to-apples). All this behind-the-scenes work is necessary for industry acceptance. As BCG noted, lack of standardization and predictable accuracy is a barrier, and regulators/customers expect validated performance and safety.
Regulatory and Security Issues
Some quantum sensors, by virtue of their capabilities, raise regulatory concerns. For instance, a quantum gravimeter that can detect hidden tunnels might be considered sensitive military equipment – governments might regulate its export like they do night-vision or radar systems. Quantum magnetometers that could detect submarines could be subject to similar controls. There’s also the question of spectrum: a quantum radar (if it emits entangled microwaves) still occupies spectrum and might need frequency licensing. And safety: high-power lasers in some sensors mean eye-safety regulations for commercial products (a LIDAR on a car must be eye-safe, which constrains its power and wavelength – quantum lidar must also obey this).
For healthcare sensors, regulatory approval (FDA, CE marking) can be lengthy – proving that a quantum MEG device is safe and effective will take clinical trials and bureaucratic processes, which are hurdles beyond just making the tech work. Moreover, training end-users (doctors, soldiers, technicians) to use these complex devices is non-trivial – workforce development is an often overlooked challenge. The quantum sensors might require new skill sets that current staff don’t have, so companies and governments will need to invest in education and training programs.
Ethical and Privacy Concerns
If quantum sensors allow seeing through walls or detecting things at a distance that were previously hidden, there could be privacy implications. A hypothetical ultra-sensitive magnetic sensor might, in the far future, pick up the magnetic signals of a person’s heart or brain from a short distance – effectively a remote lie detector or health monitor. Society would need to decide what is acceptable use. While this is not an immediate roadblock, it’s worth noting that adoption might not only be about technology but also whether we want certain capabilities freely deployed.
In summary, the road to widespread quantum sensing is challenging but navigable. Technical issues like noise and stability are being addressed by clever engineering (e.g., common-mode rejection in the gravity gradiometer removed vibrational noise ). Commercial issues like cost and complexity will likely be solved as the ecosystem matures – reminiscent of how early computers were room-sized and finicky, but decades later we have microchips everywhere. Standardization efforts are already starting (ISO and NIST have held workshops on quantum sensor standards). Regulation and talent are hurdles that require broader community and governmental action – national initiatives are indeed funding not just research, but also workforce development and industry consortia (like the Quantum Economic Development Consortium in the US) to smooth this path.
A key point is that many quantum sensors will initially complement, not outright replace, classical ones. Hybrid solutions and incremental introduction will mitigate some risk. As those early systems prove their worth (or limitations), they’ll pave the way for improved second-generation devices. Patience is needed: some devices might hit the market in a couple of years, others might take a decade or more of refinement. But the momentum is there – as evidenced by the successful prototypes and increasing investment, the community is chipping away at these challenges one by one.
A Taxonomy of Quantum Sensors
Quantum sensing is not a monolith; it encompasses a variety of sensor types leveraging different quantum systems. Here I’ll present a structured taxonomy of quantum sensors, organized by the physical platform or phenomenon they use. Keep in mind, this is by no means an “official” or a standard taxonomy. Simply my attempt at creating some order. Each category includes several subtypes with distinct applications:
Atom- and Ion-Based Sensors
These sensors use isolated atoms or ions as exquisitely sensitive measurement devices. Atoms have quantized energy levels and can act like stable “clocks” or interferometric measurements of motion or gravity. Atom/ion sensors harness the fact that we can control quantum states of atoms with extreme precision. Their challenges are the needed apparatus (lasers, vacuum) but they lead in absolute accuracy for time and low drift for inertial sensing.
Atomic Clocks: An atomic clock measures time by monitoring the frequency of a specific atomic transition (for example, the microwave transition in cesium or an optical transition in strontium). It is essentially a quantum sensor for time/frequency. Atomic clocks are the most established quantum sensors – the cesium clock has defined the second for decades. Modern optical atomic clocks (using atoms like Sr, Yb, or single ions like Al⁺) have reached uncertainties of better than 1 part in 1018. To illustrate, NIST’s F2 cesium fountain clock wouldn’t lose a second in 300 million years, and newer optical clocks improve on that by orders of magnitude. These clocks underpin GPS, telecom network timing, and fundamental physics tests. They fall under “quantum sensing” because they use quantum resonance as a reference. Atomic clocks can also act as sensors of gravitational potential (detecting tiny frequency shifts due to gravity). Portable atomic clocks are emerging – chip-scale clocks are already used in some military systems, and optical clocks are being ruggedized for field use.
Atomic Interferometers (Accelerometers & Gyroscopes): These devices use matter-wave interference of atoms to sense acceleration, rotation, or gravity. In a typical setup, laser pulses put atoms in a superposition of momentum states and then recombine them; the interference pattern depends on the acceleration or rotation (via phase shifts). Atomic accelerometers and gyroscopes measure linear acceleration and angular rotation, respectively, with extremely low drift. They form the basis of quantum inertial navigation units. For example, cold-atom gyroscopes have demonstrated rotation sensitivity that could rival fiber-optic gyros, and cold-atom accelerometers have shown the ability to hold stable with minimal drift for many hours. These sensors usually use free-falling atoms in a vacuum chamber (like a fountain or an optical cavity) and require precise laser timing. The French company Muquans (then iXblue, and now Exail) built a transportable atomic accelerometer, and the UK has tested one on a plane. As a subtype, matter-wave gravimeters (next item) are essentially atomic accelerometers oriented to measure the acceleration due to gravity.
Atom Interferometric Gravimeters and Gradiometers: A specialized atomic interferometer that measures the local gravitational acceleration (g). By dropping cold atoms and using an interferometer sequence, these devices sense gravity very precisely. They can measure the absolute value of g or, in a gradiometer configuration (two interferometers at different heights), the gradient of the gravitational field. Absolute quantum gravimeters have been used to monitor volcanoes, water table changes, and for geodesy. The quantum gradiometer demonstrated in 2019 had two clouds 1 m apart in an “hourglass” and could detect an underground tunnel via gravity gradient. Such gradiometers cancel out common noise like vibration by subtracting the signals (since both interferometers shake similarly, but only gravity gradient differs). Atom gravimeters generally require a well-controlled environment (they’re sensitive to tilt, vibration, etc.), but their low drift over time is a huge advantage over classical gravimeters which need recalibration often. These tools fall under atom-based sensors and are finding applications in geophysics and civil engineering.
Trapped Ion Sensors: Ions (charged atoms) can be trapped using electromagnetic fields and laser-cooled to form highly ordered structures (like Coulomb crystals). Trapped ions have served as superb clocks (e.g., Al⁺ ion clock) and also as electric-field sensors. A single trapped ion can act as a tiny antenna for oscillating electric fields: by observing its motional state or transition rates, one can infer the field strength. While less common than neutral atom sensors, trapped ions have the advantage of long coherence times and strong coupling to electric fields (due to their charge). They could be used for detecting THz radiation or as precision E-field meters in calibration labs. Another example: an array of trapped ions can sense forces or fields gradient across the array by changes in their vibrational mode frequencies. Trapped ions are however more lab-bound for now, since traps and vacuum setups are complex. They have mainly shone in clocks and fundamental experiments (like sensing tiny forces in tests of Newton’s inverse-square law at micrometer scales).
Photonic and Light-Based Sensors
These sensors use light – photons – and quantum states of light for sensing. Photons are great carriers of information and can be prepared in entangled or squeezed states to surpass classical limits in imaging and detection. Photonic sensors often overlap with optical technology, but exploit quantum properties of light. They often aim to surpass classical limits of resolution, detection sensitivity, or penetrating power by using entanglement, single-photon detection, or quantum interference. Many are still in experimental phase, but elements like single-photon LIDAR are already finding use.
Quantum LIDAR (Light Detection and Ranging): A LIDAR sends out laser pulses and measures reflections to create 3D maps (like “laser radar”). A quantum LIDAR uses quantum properties of the light to improve performance. One approach is to use entangled photon pairs or single-photon sensitivity to detect very faint returns. For instance, a quantum lidar system might use one photon of an entangled pair as a reference and the other as the probe that reflects off a target, similar to quantum illumination, thus extracting the target signal from noise more effectively. Another approach is using photon-counting and time-correlated techniques – essentially leveraging the particle nature of light and the ability to detect single photons with near-zero false counts. The benefit is improved range (seeing further at the same laser power) and working in poor conditions (fog, rain) by distinguishing signal photons from background via quantum signatures. Quantum lidar is useful for autonomous vehicles, drones, and terrain mapping; a photonic lidar system under development with entangled or frequency-correlated photons promises higher resolution and the ability to see through obscurants better than classical lidar. Some prototypes claim the ability to achieve required range at eye-safe power by leveraging photon counting. In short, quantum lidar is an evolving subset of lidar aiming for more precise 3D imaging using fewer photons and clever detection.
Quantum Radar & Quantum Illumination: As discussed, quantum radar uses microwave photons in quantum states (like entangled or squeezed states) to detect objects with better sensitivity or stealth-penetrating ability. The typical model is entangling a signal and idler photon, sending the signal (microwave or possibly millimeter-wave) at the target, and keeping the idler (often a lower frequency or optical photon) for reference. At the receiver, a joint measurement of the reflected signal and the stored idler can confirm a target’s presence with fewer false alarms. Quantum radar could, in theory, detect targets with a much lower power emission (meaning it could be covert and also less prone to being fooled by jamming). Laboratory demonstrations have been in optical regimes at short distance. Another variant is using squeezed-state transmitters to reduce transmitter noise and improve detection of slow-moving objects in clutter (a concept called quantum-enhanced noise radar). While no full-scale quantum radar exists yet, research continues, combining photonic techniques (entangled photon generation, parametric amplifiers) with classical radar architecture. If realized, it would be a game-changer for surveillance – e.g., seeing through chaff or detecting very small radar cross-section objects. In taxonomy terms, I include it under photonic sensors as it fundamentally uses properties of photon fields (like entanglement) for sensing.
Ghost Imaging Systems: Ghost imaging, as described earlier, involves forming images using correlated light beams. A quantum ghost imaging setup uses entangled photon pairs: one beam (the “signal”) illuminates the object and is collected by a single-pixel (bucket) detector with no spatial resolution; the other beam (the “idler”) doesn’t hit the object but goes directly to a high-resolution camera. Surprisingly, an image of the object emerges on the camera by correlating which photon arrivals at the camera coincide with detections at the bucket detector. Essentially, the camera sees a random pattern, but by knowing when the bucket detector clicked, one can sift the camera data to build the image. The quantum entanglement or at least strong correlation is key. Ghost imaging can work even if the object’s beam is very weak or scatters widely, as long as the coincidences can be identified. This technique has been demonstrated to see through turbulence and partial obstructions. In practice, true entangled-photon ghost imaging has low rates, but “computational ghost imaging” and classical correlated source ghost imaging have also been developed, which mimic some benefits without full quantum resources. Nonetheless, ghost imaging is a paradigm for sensing where the sensing and reference portions are separate, leveraging quantum correlations. It has potential in biomedical scanning (using penetrating infrared light on the sample and detecting with visible light on the reference arm) and in remote sensing (creating images with very low light flux on the subject).
Plasmonic Quantum Sensors: Plasmons are collective oscillations of electrons at metal surfaces, used in highly sensitive classical sensors (like surface plasmon resonance (SPR) sensors for detecting biomolecules). Quantum plasmonic sensors marry these with quantum light or detect single-plasmon events. For example, using squeezed light or entangled photons in an SPR setup can enhance the precision of measuring refractive index changes (which occur when biomolecules bind to a sensor surface). Also, plasmons can confine light to nanoscale volumes, enabling interactions with quantum emitters (like quantum dots or NV centers) that wouldn’t happen with normal light due to diffraction limits. Quantum plasmonic sensing might involve single-plasmon generation and detection to push sensitivity to the level of single molecules. A practical benefit is improved signal-to-noise and potentially lower detection limits for chemical/biological sensing. This is an emerging category, blending nanophotonics and quantum optics; early reviews (e.g., a 2018 Chemical Reviews article) outline how quantum techniques (photon antibunching, squeezed states) can enhance plasmonic sensors’ performance. As a category, it’s a subset of photonic sensors specialized for chemical sensing at surfaces and nano-interfaces.
Magnetometry
Magnetometry deals with measuring magnetic fields. Quantum magnetometers are among the most sensitive sensors of any kind, capable of detecting fields a million times weaker than Earth’s magnetic field. SQUIDs set the benchmark for sensitivity, OPMs are rapidly becoming the practical choice for many applications due to not needing cryogenics, and NV centers offer high resolution and novel use cases. Magnetometers are central in healthcare, defense, and materials analysis, so improving them has wide impact. Different quantum effects are exploited to measure magnetism:
SQUID Magnetometers: The Superconducting Quantum Interference Device (SQUID) is a hallmark quantum sensor that’s been in use since the 1960s. It consists of a superconducting loop with one or two Josephson junctions. The magnetic flux through the loop causes quantum interference of the superconducting wavefunction, resulting in an oscillating electrical output. SQUIDs can detect changes in magnetic flux on the order of $$10^{-15}$$ Tesla (a millionth of a billionth of Earth’s field) – sensitive enough for biomagnetic signals like the brain’s fields. They are used in magnetoencephalography (MEG) and geophysics. However, SQUIDs require cryogenic cooling (liquid helium or liquid nitrogen for high-$$T_c$$ SQUIDs) and magnetic shielding. They were essentially the first commercial quantum sensors. Their high cost and complexity spurred the search for alternatives. Still, they represent the pinnacle of magnetic sensitivity in many scenarios. For example, multi-SQUID MEG systems can detect the tiny magnetic fields from neural currents to localize brain activity for epilepsy patients. As quantum devices, SQUIDs rely on quantum tunneling and flux quantization – phenomena with no classical counterpart. The downside: bulky, expensive (due to cryogenics), and need careful shielding. But they set the standard that newer quantum magnetometers aim to match at room temperature.
Optically Pumped Magnetometers (OPMs): OPMs use atomic vapor (like rubidium, cesium, or helium gas) and exploit the precession of atomic spins in a magnetic field. By optically pumping (using a laser to align spins) and then detecting how the spins process (using another laser beam’s polarization rotation or fluorescence), the magnetic field can be inferred. OPMs have achieved sensitivities on par with SQUIDs in the best cases, without cryogenics. A specific type, the SERF (Spin-Exchange Relaxation-Free) magnetometer, operating in near-zero magnetic field, holds the record for sensitivity ~0.5 fT/√Hz, rivaling SQUIDs. OPMs can be small (a few cm cells) and operate at room or moderate temperature. Some require heating the vapor cell (to increase atom density) to ~150°C and use magnetic shielding to reach fT sensitivity. The best OPMs now enable wearable MEG as discussed, and are also used in portable magnetometers for field measurements. Their advantages are cost and size compared to SQUID – no need for expensive cryogens – and they can be made fairly robust. They are used in applications from brain imaging to detecting unexploded ordnance. Companies and labs are actively improving OPM bandwidth and stability. Because they are quantum devices (the measurement relies on quantum spin precession and optical readout via atomic energy levels), they are susceptible to quantum shot noise and spin-projection noise, which researchers mitigate via techniques like spin-squeezing the atomic ensemble (a demonstration by MIT in 2020 improved magnetometer SNR by squeezing the atoms). OPMs represent a very successful translation of quantum physics (optical pumping invented in the 1950s by Kastler) into practical sensors that today compete with the long-dominant SQUID.
NV-Center Diamond Magnetometers: The nitrogen-vacancy (NV) center in diamond is a point defect that behaves like an isolated quantum spin which can be manipulated and read out with light even at room temperature. NV centers have an electron spin that can be initialized with a laser and read out via its fluorescence, and this spin’s resonance depends on the local magnetic field (Zeeman shift). By measuring changes in the NV’s electron spin resonance (ESR) frequency, one can deduce the magnetic field. NV magnetometers can be highly sensitive and have extreme spatial resolution – a single NV can sense fields at the nanometer scale (essentially acting as a nanoscale magnetometer). In ensemble (many NVs in a diamond) they can reach sensitivities in the picotesla range with averaging. NV sensors have been used to image magnetic domains in materials, map currents in integrated circuits (by scanning an NV tip over a chip to see the magnetic field from currents), and even to detect neuronal action potentials in marine worm neurons in a lab (an early bio-sensing feat at microscale). While NV magnetometers currently may not beat SQUIDs in raw sensitivity in a head-to-head, their ability to work at room temperature and at tiny scales opens new applications. They also double as electrometers and thermometers (NV spin levels are affected by electric fields and temperature too). Companies are building NV magnetometer microscopes for the semiconductor industry to use in failure analysis – essentially quantum-based MRIs for electronics. NV centers have also been integrated into tiny needles for scanning probe magnetometry, achieving nanometer resolution magnetic field maps. The challenge for NV sensors is that single-spin sensitivity requires good optical collection and often long averaging, and ensemble NV sensitivity can be limited by diamond quality and inhomogeneous broadening of the spins. Active research is making better diamond material (isotopically pure C-12 diamond) to reduce decoherence. NV magnetometers are a prime example of a solid-state quantum sensor that leverages techniques from quantum computing (coherent spin control) for sensing tasks.
Other Solid-State Quantum Magnetometers: Beyond NVs, there are other quantum defects and materials for magnetometry. Examples include phosphorus donors in silicon, quantum Hall magnetometers, or emerging 2D materials where electron spins can be used for sensing. There are also quantum spin liquids or exotically, using quantum superconducting qubits as magnetometers (e.g., a transmon qubit’s frequency shifting with field). These are mostly in research phase. Another notable type is fluxgate magnetometers – although largely classical, some advanced designs blur into quantum domain by using non-linear magnetic cores and could be enhanced by quantum readout. However, fluxgates are less sensitive than the ones above, so quantum improvements are focused on the types listed.
RF and Microwave Quantum Sensors
Quantum techniques can be applied to sensing electric fields, electromagnetic waves, and photons in the radiofrequency (RF), microwave, and millimeter-wave domains. Traditionally, antennas and electronic receivers handle these, but quantum sensors can provide absolute calibration, broad bandwidth, and high sensitivity in ways classical devices cannot. RF and microwave quantum sensors are expanding how we measure electromagnetic fields. They bring atomic precision and quantum-limited noise performance to frequencies from kHz to THz. This category is rapidly developing, especially thanks to Rydberg atom research and superconducting quantum circuits. The goal is often a more absolute and sensitive measurement of fields, which is valuable in communications, radar, and standards.
Rydberg Atom RF Sensors: Rydberg atoms (atoms excited to very high principal quantum number) have exaggerated electromagnetic responses. They can be used as all-in-one RF field probes. When a vapor of Rydberg atoms is exposed to an RF field, the field causes transitions between Rydberg states (or shifts in their energies) that can be optically detected (via electromagnetically induced transparency or fluorescence). By scanning a laser over a Rydberg resonance and observing changes, one can measure the amplitude and frequency of the RF field. These sensors cover frequencies from ~kHz up to THz by selecting appropriate atomic transitions. A major advantage is that they are self-calibrated – the sensitivity is given by atomic constants, linking volts per meter to frequency via Planck’s constant. NIST has demonstrated Rydberg sensors picking up AM/FM radio, Wi-Fi, Bluetooth signals and directly demodulating them. They’ve shown detection from 0.1 to 100 GHz with one setup, which is unprecedented bandwidth in a single device. They also achieved angle-of-arrival sensing by using two vapor cells and detecting phase differences. The Rydberg receiver showcased in 2023 could receive real communications signals over long distance, with extraordinary sensitivity across HF to SHF bands. Another interesting feature: Rydberg sensors can have high dynamic range and are less prone to saturation by strong signals (they inherently only respond to the absolute field value in a known way). These sensors are still being refined – issues include laser noise and the need for dual lasers (to prepare and probe the Rydberg state). But they are extremely promising for applications like spectrum monitoring, EW (electronic warfare), and even as exotic as replacing antenna arrays with atomic vapor cells. They represent a new kind of sensor that directly converts RF fields to optical signals (the readout laser gets modulated by the RF via the atoms). Given they’re based on atoms, they align well in a quantum sensing taxonomy. We can think of them as the RF analog of atomic clocks: where a clock gives frequency from time, a Rydberg sensor gives field strength from atomic resonance. Notably, they offer traceable measurements of field strength, which is valuable for metrology (calibrating other instruments). The U.S. Army and NIST have been actively advancing this (as cited earlier, the Army illustration shows it detecting various communications bands ). Rydberg sensors are a standout example of an emerging quantum sensor that didn’t exist a decade ago.
Superconducting Quantum RF Sensors: Superconductors appear again in RF sensing beyond SQUIDs. One example is the Kinetic Inductance Detector (KID) – a superconducting resonator that can absorb single millimeter-wave or sub-mm photons, changing its impedance. KIDs are used in astronomy (for cosmic microwave background detection) as they can cover large detector arrays and are simple to read out with microwave tones. They are essentially quantum in that they rely on superconductivity and can be sensitive to single quanta of energy. Similarly, Josephson parametric amplifiers (JPAs) are used as near-quantum-limited amplifiers for microwaves (e.g., in reading out superconducting qubits in quantum computers). As sensors, JPAs can amplify tiny RF signals with almost no added noise (just at the standard quantum limit). This is crucial for applications like axion dark matter detectors (like the ADMX experiment) where a JPA amplifies a feeble microwave signal presumably generated by axions converting in a magnetic field. These superconducting RF sensors do require cryogenics, but they provide capabilities unmatched by conventional electronics (you can’t classically amplify with as low noise as a JPA provides, since a classical amplifier adds at least half a photon of noise at best, while a JPA approaches that quantum half-photon limit). Another device, the quantum upconverter, can convert microwave signals to optical domain via, say, an electro-optic crystal or a mechanical oscillator; while not purely “sensors,” these help interface quantum sensors to existing telecom optics.
Spin-Based RF Sensors: NV centers and OPMs can also function as RF sensors if configured properly. For instance, NV centers can detect AC magnetic fields in the MHz to GHz range by an effect called spin-locking or by measuring Rabi oscillation frequency shifts. This could be used for NMR spectroscopy on tiny samples (the NV acting like a micro-coil). Similarly, atomic vapor cells can detect radio-frequency fields via AC Zeeman effects or by using Rydberg states (as above). So there is cross-over between magnetometry and RF sensing: an oscillating magnetic field is an RF field, after all.
Quantum Radar Frequency Sensors: This includes gadgets like quantum spectrum analyzers (the Rydberg cell is one example). Another concept is using entangled photons to sense RF – for example, doing interferometry with microwaves using optical interferometers via transducers (though that’s complex). Also, the idea of a “quantum antenna” – like using a qubit or quantum circuit as an antenna for specific frequencies, leveraging its discrete energy levels. One could imagine a superconducting qubit tuned to a certain frequency that “flips” when it absorbs a photon of that frequency, effectively counting photons in that band (quantum counting instead of classical power measurement). These are not mainstream yet, but are studied in quantum computing contexts (where stray microwave photons are bad for qubits, so they actually have photon counters to detect them).
Quantum Thermometry and Other Niche Sensors
Quantum principles can enhance sensors for various other physical quantities – some of these are emerging or niche, but illustrate the breadth of quantum sensing.
Quantum Thermometers: Temperature can be sensed on very small scales by quantum systems whose properties change with temperature. One example is an NV center in diamond – its fluorescence spectrum slightly shifts with temperature, allowing it to act as a nano-thermometer inside cells or materials, with sensitivity on the order of milliKelvins at nanometer resolutions. Another example is using two energy levels of an ion with different sensitivities to temperature (one might be a highly temperature-dependent transition). By measuring their frequency difference, temperature can be deduced. At ultra-cold temperatures, superconducting devices (like the Johnson noise in a resistor measured by a SQUID) can do primary thermometry. Quantum thermometry is useful in situations like monitoring the temperature in a living cell, or the local temperature in an electronic circuit hotspot, where classical sensors (thermocouples, etc.) are too large or perturbative. The quantum advantage is often the ability to sense temperature changes at extremely small scales or low magnitudes. For instance, researchers put quantum dots in tissues to measure heating from therapies, or used trapped ions to detect minuscule temperature shifts indicating heating in ion traps. These sensors typically cover the 1 K to 400 K range (for NV, roughly 0–100°C with high sensitivity, and for superconducting thermometers, microKelvin to a few Kelvin). Practical adoption is still mostly in research, but in the future, one could see quantum thermal sensors calibrating the next generation of chip cooling systems or in climate research instruments.
Quantum Pressure and Force Sensors: Some quantum sensors measure force or pressure by converting them into frequency shifts. One approach is an optomechanical sensor: a tiny mirror or membrane in a cavity can be pushed by pressure or force, shifting the resonance frequency of the cavity that’s read by a laser. If the mechanical element is in a near-quantum ground state (achieved by cooling or optical trapping), it can detect forces at the limit of the Heisenberg uncertainty principle for the momentum and position of that object. This has been used to detect forces as small as $$10^{-18}$$ N (attonewton scale), relevant in AFM (atomic force microscopy) or detecting gravity forces between small masses. Another example: Rydberg atoms in a vapor can act as mini barometers – the collision rate (and thus spectral line width) of the Rydberg transition depends on gas density/pressure, so by reading that you get pressure. SQUID-based devices can measure pressure by detecting tiny changes in a diaphragm coupled to a superconducting circuit (merging mechanical and quantum). While classical pressure sensors are quite good, quantum ones might be needed for extreme sensitivity or ranges (like ultra-high vacuum measurement beyond what ion gauges can do, or minute pressure changes in the atmosphere for science).
Optomechanical Sensors: This overlaps with force, but deserves mention: optomechanics involves coupling light to mechanical motion. By using laser light, one can cool, measure, and even prepare mechanical oscillators in quantum states. Optomechanical sensors can measure displacement (a tiny mirror’s movement) to 1e-20 m/√Hz levels (related to gravitational wave tech). They can also detect forces or masses (if a tiny mass is attached to the oscillator, its presence shifts frequency). One interesting device is a microcantilever with an embedded qubit (like an NV center) that acts as a quantum readout of the cantilever’s vibrations – used to sense magnetic forces from single spins (as in MRFM – magnetic resonance force microscopy). In LIGO, the 40 kg mirrors are essentially quantum optomechanical sensors for gravitational waves; at the other extreme, nanogram-scale oscillators in labs are being used to weigh single viruses or nanoparticles, with quantum techniques potentially improving their resolution.
Quantum Acoustic Sensors: Similar to optomechanics but with sound – devices like superconducting qubits that detect single phonons (quantum of sound). These could serve as extremely sensitive microphones or ultrasound detectors. For example, detecting individual quanta of vibration in crystals (useful in quantum info as well but also for sensing minute acoustic signals).
Other Novel Sensors: There are many creative quantum sensor ideas. To list a few: Quantum GPS – not exactly a sensor, but using entangled clocks or sensing signals to improve GPS accuracy or spoof resistance. Quantum gravitometers – akin to gravimeters but maybe using entangled particle pairs to sense curvature (still hypothetical). Chemical sensors using quantum algorithms – e.g., a small quantum computer evaluating molecular spectra could be seen as a sensor for chemical presence (blurring computing and sensing). Quantum imaging sensors in cameras – single-photon avalanche diodes (SPADs) array in smartphone cameras are arguably on the fringe of quantum sensing, since they detect single quanta of light (though they operate classically, their existence owes to quantum understanding of photodetection).
In a structured sense, we categorize by platform, but indeed some sensors don’t fit neatly because they combine systems (e.g., optomechanical involves light and mechanics, NV involves solid-state spin and optical, etc.). The taxonomy above covers the major groupings recognized in literature:
- Atomic/ionic (time, inertial, gravity)
- Photonic/imaging (lidar, radar, quantum cam)
- Magnetometers (SQUID, NV, OPM)
- RF/microwave (Rydberg, quantum circuits)
- Others (thermo, pressure, etc.)
Each type has unique strengths: atomic systems excel in stability and accuracy, photonic in speed and resolution, magnetometers in sensitivity to fields, etc. And they often complement each other – for instance, atomic sensors might use photonic readout; NV centers combine solid-state and optical; SQUIDs detect magnetic flux for both magnetic and (with attachments) physical quantities like pressure or inertia.
As quantum sensing technology matures, we may see hybrid sensors (combining categories). Already there are proposals for hybrid magneto-gravimetric sensors (measuring gravity and magnetic field together for geophysics). The taxonomy helps understand the landscape, but future devices might be multi-modal.
Future Prospects and Conclusion
Quantum sensing is on the cusp of transitioning from laboratory curiosity to a mainstream technological tool. Looking ahead, several exciting developments and trends are likely to shape the future of this field:
Integration and Miniaturization: The next decade should see quantum sensors shrink from room-sized setups to chip-scale devices. Just as we have integrated circuits, we will develop integrated quantum sensors – combining lasers, photonics, and even vacuum chambers on a chip. Research is already moving towards wafer-scale fabrication of vapor cells and integrated photonic circuits for routing light to atoms or NV centers. One can imagine a single chip that contains an array of quantum sensors (for example, a tri-axial quantum accelerometer/gyroscope unit with atomic beams in different orientations). This integration will reduce cost and power consumption dramatically, opening the door to putting quantum sensors in everyday devices. Early examples like NIST’s chip-scale clock show it’s feasible to bring quantum devices to the micro-scale; similar efforts will yield chip-scale magnetometers (some OPMs are already palm-sized) and mini atomic interferometers. With size reduction, power requirements will drop too – ultimately perhaps allowing battery-operated quantum sensors. This paves the way for mobile platforms: drones carrying quantum gravity sensors to survey disaster sites, or handheld medical scanners with quantum magnetometers for point-of-care diagnostics.
Quantum Sensor Networks: Today, we often operate one sensor at a time. In the future, connecting multiple quantum sensors could unlock new capabilities. Entangled sensors distributed in a network can act in unison – for instance, an array of entangled magnetometers could cancel common noise and pick up extremely subtle correlated signals (useful in global searches for exotic physics). Even without entanglement, networks of quantum sensors can provide unprecedented coverage – e.g., a worldwide network of atomic clocks and gravimeters monitoring the Earth in real-time for gravitational changes (this could detect earthquakes or volcanic events via gravity signals slightly faster than seismic waves travel). The term “quantum IoT” is sometimes used for the vision of many quantum sensors deployed and communicating. Entanglement distribution (via quantum communication links) could allow, for example, a baseline of two telescopes far apart to perform quantum-enhanced interferometry, improving astronomical imaging resolution beyond classical limits. Also, clock networks with entanglement could achieve time synchronization impossible classically, benefiting GPS-type systems or trading networks (where nanosecond timing can matter). In essence, the future may hold webs of quantum sensors that collaboratively sense large-scale phenomena – a sort of quantum supersensor composed of many nodes.
Synergy with Quantum Computing and Quantum Communication: The rise of quantum computers and quantum communication infrastructure can feed into sensing advances. For one, quantum computers themselves need excellent qubits – which are basically sensors (a qubit is very sensitive to its environment, which is usually a bane for computing but a boon for sensing if harnessed). Techniques developed to protect qubits (quantum error correction) might be applied to sensors to correct their errors and noise, leading to fault-tolerant quantum sensing. Conversely, a quantum computer could be used to process quantum sensor data optimally – for example, using quantum algorithms to extract patterns from a set of entangled sensor measurements that classical processing might miss. Quantum communication links (fiber or free-space) being built for quantum key distribution (QKD) could double as channels to send entangled states or timing signals for sensor networks. There is a vision of a Quantum Internet which not only transmits qubits for computation/communication but also connects sensor devices – enabling things like baseline-entangled telescopes or distant clock comparisons in real time. Another synergy: quantum computers might require precise calibration of fields and forces – quantum sensors will be embedded in quantum processors (indeed, something like a JPA amplifier in a quantum computer is a quantum sensor for certain modes). This cross-pollination means advances in one quantum domain often benefit others.
New Modalities and Exotic Applications: As creativity meets technology, we’ll likely see quantum sensors in places we haven’t imagined. Perhaps quantum sensors will enable detection of elusive signals like cosmic high-frequency gravitational waves, or minute traces of environmental pollutants via advanced spectroscopy. They could play roles in emerging fields like quantum biology – trying to sense quantum effects in living organisms (for instance, verifying if entanglement plays a role in bird navigation by sensing tiny magnetic changes in chemical reactions inside cells). In space, quantum sensors might be placed on other planets for science (an optical clock on Mars could test gravitational redshift in a new way, or a quantum gravimeter on the Moon might map its interior precisely). If dark matter or other new physics is found via quantum sensors, that would be a monumental scientific achievement with Nobel-level impact – and quite possible given experiments like atomic clocks and magnetometers are pushing into parameter spaces unexplored before.
Commercial Breakthroughs and Ubiquity: On the commercial side, once a few quantum sensor products demonstrate clear value (perhaps a quantum gravimeter that saves millions in a construction project by mapping voids, or a quantum medical scanner that diagnoses a condition non-invasively that previously required surgery), demand could rapidly increase. Economies of scale would then kick in, further lowering costs, leading to a virtuous cycle. It’s conceivable that in 20 years, quantum sensors will be as ubiquitous as GPS chips are today – not necessarily recognized as “quantum” by end users, but quietly embedded in devices and systems. For example, every data center might have a rack of quantum clocks to time operations; every hospital might have a quantum brain scanner and quantum molecular analyzer; every airplane might carry a quantum inertial backup to GPS; and smartphones might incorporate quantum-enhanced magnetic and inertial sensors for navigation and AR (augmented reality) precision. When technology fades into the background of everyday life, you know it’s been successfully absorbed – and that could happen with quantum sensing.
Overcoming Today’s Roadblocks: The challenges we outlined will be addressed one by one. There’s a strong sense of déjà vu with other tech revolutions. Compare quantum sensing’s state now to, say, computing in the 1940s: bulky, expensive, but clearly transformative for those who could use it. Then research and industry collaborated to miniaturize and mass-produce it, and now microprocessors are everywhere. We can expect a similar trajectory. Governments are funding “quantum foundries” to fabricate quantum devices at scale, which will help overcome scalability issues. Standardization bodies are already discussing quantum sensor benchmarks, which will smooth adoption. And as more young engineers and scientists are trained in quantum tech, the talent pool limitation will ease.
In conclusion, quantum sensing is poised to augment and in some cases revolutionize how we measure the world. Its unique ability to leverage fundamental quantum phenomena – superposition, entanglement, and more – means it can achieve what was once thought impossible: detecting the seemingly undetectable. This field stands at a nexus between quantum physics and the real world, turning esoteric quantum effects into practical tools. As the technology matures, we will gain new eyes and ears (and noses and fingers, metaphorically) for science and industry. We’ll “see” underground structures without digging, “hear” the whispers of neuronal electric currents without probes, “feel” the drift of time in different gravitational potentials, and maybe even sniff out particles from beyond the Standard Model.
The journey from fundamental physics labs to deployed quantum sensors is well underway. Each year, lab breakthroughs make headlines – and then quickly transition into prototypes (the tunnel detection, the brain scanner helmet, the atomic radio receiver, to name a recent few). In a way, quantum sensing brings the quantum revolution full circle: quantum theory began by explaining measurements (like why heated objects glow with certain spectra), and now it’s being used to improve measurements. For a tech-savvy observer, the message is that quantum sensing is the quantum technology likely to impact a broad array of industries in the near term – possibly even sooner and more profoundly than quantum computing or communication – because it tackles the here-and-now challenges of measurement and detection.
The coming years will be crucial. If current trends continue, we’ll witness quantum sensors leaving the controlled confines of labs and becoming robust in the field. The ultimate success will be when users no longer even remark that a sensor is “quantum” – they’ll simply appreciate that it performs remarkably well. Much like we use GPS without thinking about atomic clocks and relativity corrections, future technicians might use a handheld quantum analyzer to image pipes in a wall or a doctor might run a quantum scan of a patient’s heart, all without fanfare – it will just be part of the toolkit.