Quantum Radar: The Next Frontier of Stealth Detection and Beyond
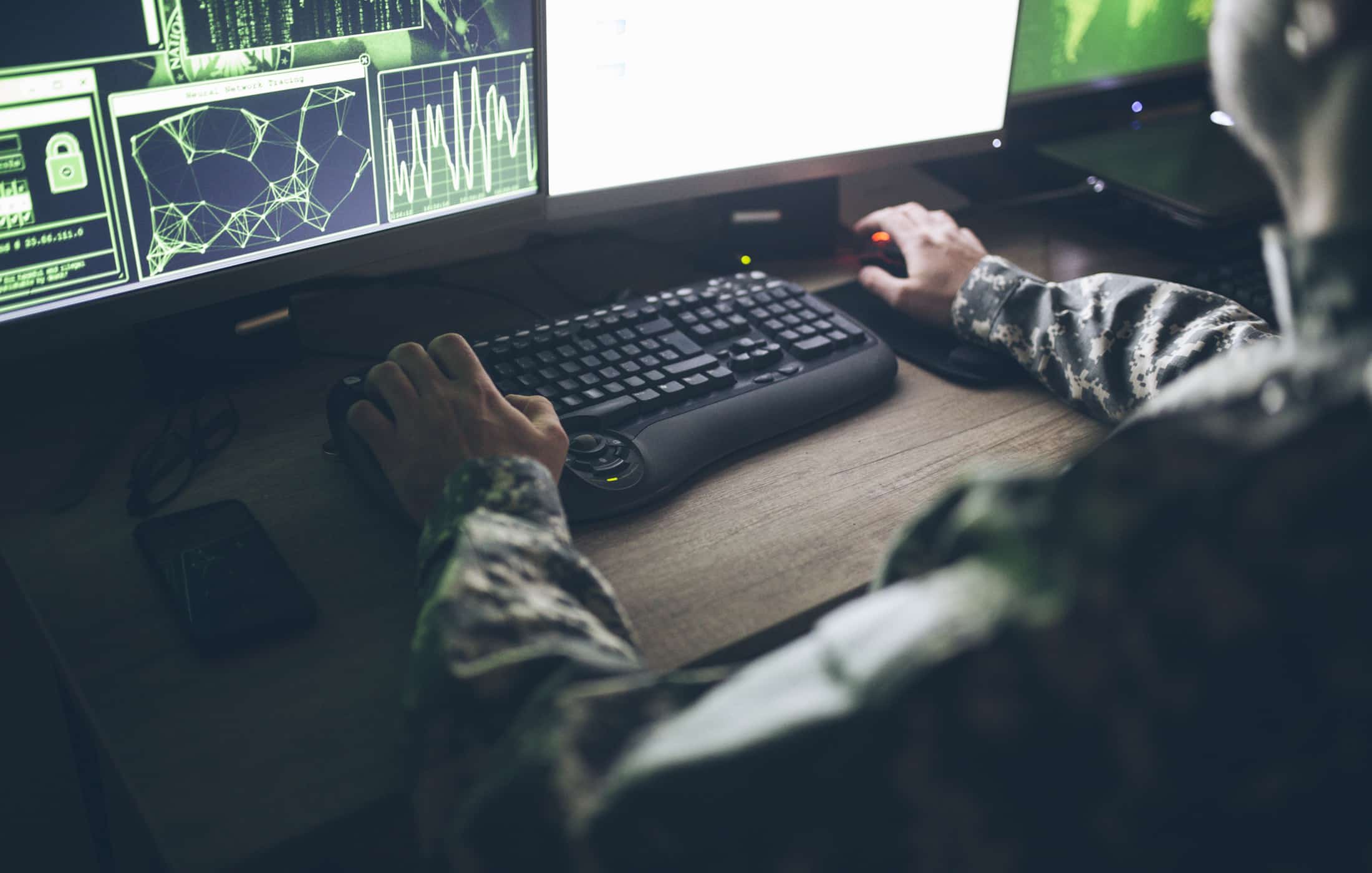
Table of Contents
Quantum radar is an emerging technology that applies the mind-bending principles of quantum mechanics to the field of radar sensing. In theory, it promises detection capabilities beyond the reach of conventional radar, potentially piercing the invisibility of stealth aircraft and opening new possibilities in sensing. From its conceptual origins in quantum physics labs to recent experimental prototypes, quantum radar has become a hot topic in defense tech circles and beyond. In this article, we explore what quantum radar is, how it works, its development history, key experiments, applications in military and civilian domains, its current status and challenges, comparisons with classical radar, the security implications of its adoption, the role of AI in enhancing it, and what the future might hold for this quantum-powered sensor.
What is Quantum Radar?
Quantum radar is essentially a radar system that exploits quantum-mechanical phenomena—such as entanglement and other non-classical correlations—to detect objects with greater sensitivity or in conditions where classical radars struggle. In a traditional radar, a transmitter sends out electromagnetic waves (often microwaves or radio waves) and a receiver listens for any reflection (echo) from a target. The strength and timing of that echo reveal the target’s distance and perhaps its size or speed. Quantum radar, by contrast, doesn’t just send out a generic signal; it sends out quantum entangled signals that are intrinsically linked to a reference signal kept at the receiver. Because of this special link, a quantum radar can know if a faint return signal is indeed from its own transmitter or just random noise, potentially allowing it to detect targets that would be lost in the noise by a classical radar.
At the heart of most quantum radar designs is the concept of quantum entanglement. Entangled particles (or photons, in this case) have correlated properties such that measuring one instantly influences the state of the other, no matter the distance between them. A quantum radar system might generate a pair of entangled photon streams: one stream is the signal beam that is emitted outwards to probe for targets, while the other is the idler beam that is retained at the radar site as a reference. If a target is present, some of the signal photons will bounce off it and return, albeit very weakly. When the return is combined with the stored idler photons, the system performs a joint measurement comparing the two. Thanks to the initial entanglement, the returning photons (if any) can be distinguished from background noise with higher confidence than an un-entangled classical signal of equal intensity. This concept is known as quantum illumination – it was shown theoretically in 2008 that entangled light can yield a significant detection advantage in noisy, lossy conditions.
Several quantum phenomena enable quantum radar to surpass conventional limitations. Quantum illumination, as described above, takes advantage of the fact that even when entanglement is broken by transmission loss, some residual correlations remain that no purely classical system can mimic. This allows a properly designed quantum radar to detect a low-reflectivity object in a bright background (like a stealth plane against sky clutter) with far fewer false alarms. Another useful phenomenon is squeezing, where the uncertainty (noise) in one property of light is reduced at the expense of another (as allowed by the Heisenberg uncertainty principle). In practice, generating a two-mode squeezed vacuum state produces entangled microwave photons; such states form the basis of some quantum radar schemes, sometimes called “quantum two-mode squeezed radar.” By using squeezed or entangled states, quantum radars can, in theory, beat the sensitivity and noise floor limits of classical radars operating at the same power. In short, while a classical radar passively waits for a weak echo to emerge from noise, a quantum radar actively labels its photons with quantum correlations, giving it a subtle “lock-and-key” way to discern signal from noise.
History and Evolution
The idea of blending quantum physics with radar dates back only a couple of decades. One early conceptual step came in the mid-2000s: in 2005, engineers at Lockheed Martin (a major U.S. defense contractor) proposed a “quantum radar” design and later filed a patent, aiming to improve resolution and detail beyond classical radar limits. However, that early design did not clearly demonstrate a true quantum advantage – it was more an exotic radar concept than a proven quantum-enabled system. The true theoretical foundation of quantum radar was laid in 2008, when MIT professor Seth Lloyd and collaborators introduced the formal concept of quantum illumination. Lloyd’s paper showed that using entangled photon pairs (signal and idler) and a joint detection strategy could, remarkably, yield an exponential improvement in the signal-to-noise ratio for detecting objects buried in thermal noise. This was soon followed by related work using continuous-variable entanglement (Gaussian states) by researchers including Jeffrey Shapiro, Stefano Pirandola, and others, which confirmed a more practical 6 dB advantage in error probability using entangled light over the best classical scheme under equal conditions. These papers firmly established the enticing theoretical promise of quantum radar: dramatically better detection of faint targets in noisy environments by exploiting quantum correlations.
In the 2010s, the focus shifted to turning theory into practice. A key theoretical advance came in 2015 when an international team proposed a microwave-frequency quantum radar model. They described how one could entangle a microwave signal beam with an optical idler via a special electro-optomechanical converter, send the microwave beam at a target, then convert the return back to optical to interfere with the idler. This approach essentially adapted quantum illumination to radar’s natural microwave domain. Around the same time, defense research organizations started paying attention. The U.S. DARPA and other agencies launched programs in quantum sensing, exploring ways quantum tech might improve detection of radio-frequency signals (for example, DARPA’s Quantum Apertures program sought quantum-enhanced receivers using Rydberg atoms, a different quantum technique). Meanwhile, in China, significant resources were poured into quantum radar research, with the state-owned China Electronics Technology Group Corporation (CETC) reportedly building a experimental quantum radar by 2016. CETC claimed a breakthrough in entangled-photon radar that could detect targets at up to 100 km, grabbing headlines worldwide with talk of a “stealth-detecting radar.” (We’ll examine those claims and skepticism around them shortly.)
By the late 2010s, actual prototypes were being demonstrated in the lab. In 2018, researchers at the Institute for Quantum Computing (University of Waterloo, Canada) and Defence Research and Development Canada built a prototype “quantum-enhanced noise radar.” In a head-to-head test, they showed it could detect a target about 10 times more effectively than an equivalent classical radar in the same noisy conditions. This was achieved by generating entangled microwave photons with a superconducting device and comparing performance to a classical random signal; the quantum version showed a clear advantage when signals were extremely weak. The following year, in 2019, another milestone was hit: a team led by Shabir Barzanjeh and Johannes Fink (then at the Institute of Science and Technology Austria) demonstrated a microwave quantum radar prototype operating at millikelvin temperatures. Their device used entangled two-mode squeezed microwaves to detect low-reflectivity objects at room temperature, validating that quantum illumination works in practice, at least at short range. These experiments, published in journals like Science Advances and Applied Physics Letters, were crucial in moving quantum radar from theory toward reality.
On the government side, the late 2010s saw a mix of excitement and cautious evaluation. In China, the efforts accelerated: by 2018, Chinese researchers announced improvements in their quantum radar’s coherence range and talked about deploying such radars on high-altitude airships or drones to track ballistic missiles and satellites from the upper atmosphere. China’s National Laboratory for Quantum Information Sciences, a $10 billion initiative in Hefei, has made quantum sensing (including radar) a strategic focus. Western defense analysts took note; by 2020, the U.S. and its allies were studying the feasibility of quantum radar. A U.S. Air Force-commissioned MIT Lincoln Laboratory study in 2020 concluded that while quantum radar is based on sound physics, it may have “low potential” for long-range use with current technology. Nonetheless, funding for quantum technology has continued to grow under programs like the U.S. National Quantum Initiative (authorized in 2018) which explicitly aims to keep American research at the cutting edge. In short, the past decade took quantum radar from a speculative idea to a demonstrated principle, all while nations launched quantum programs to assess how soon – or whether – it could become a game-changing sensor in the real world.
Key Papers and Experiments
Because quantum radar lies at the intersection of quantum physics and engineering, its progress has been marked by a series of influential papers and experiments bridging those fields. The foundational theoretical paper was Seth Lloyd’s 2008 work on quantum illumination, which showed entanglement could dramatically improve target detection in noisy environments. In the same year and shortly after, complementary analyses by Jeffrey Shapiro and colleagues at MIT fleshed out the concept, confirming that even if the environment destroys the entanglement, the initial quantum correlations can still confer a detection advantage. These early papers are often cited as the birth of quantum radar theory, establishing benchmarks like the 6 dB error-exponent improvement and outlining how an optimal quantum receiver would work.
Fast forward to 2015, and we see a pivotal theoretical proposal by Stefano Pirandola, Barzanjeh, and others, who described Gaussian quantum illumination applied to microwaves. This work is notable for suggesting a practical architecture: generate entangled optical photons, convert one to a microwave signal, and after reflection convert it back to optical for joint detection with the retained optical photon. It essentially merged quantum optics with radar engineering, and set the stage for building a prototype. Indeed, one of the key experimental papers followed in 2019 when C.W. Sandbo Chang et al. (Christopher Wilson’s group) published “Quantum-Enhanced Noise Radar” in Applied Physics Letters. In that experiment, the team entangled pairs of microwave photons at ~5 GHz and demonstrated detection of a test object in a noisy background, outperforming a classical noise radar by an order of magnitude in sensitivity. This was the first experimental validation that quantum illumination can beat classical in a real microwave radar scenario. Another influential experimental paper soon after was Shabir Barzanjeh et al. (2020) in Science Advances, which reported a quantum radar prototype detecting room-temperature objects using entangled microwaves produced at cryogenic temperatures. This experiment didn’t reach long distances, but it proved that the quantum advantage survives the leap from theory to hardware, at least over a few meters.
In addition to these, several other papers have shaped the discourse. Jeffrey Shapiro wrote a 2020 overview titled “The Quantum Illumination Story” (IEEE A&E Systems Magazine) recounting the development and nuances of the theory. Researchers have also explored variations like quantum two-mode squeezing radar (which forgoes joint measurement, simplifying the receiver) and published analyses comparing it to classical noise radar. On the applications side, Chinese scientists Li and Wang et al. published descriptions of their quantum radar approach, claiming the ability to measure not just position but other properties of a target, and arguing its immunity to spoofing. Furthermore, defense-related white papers and reports – e.g., a 2019 Swedish Defence Research Agency report and a Canadian Department of National Defence study – have been written to survey quantum radar’s potential. These often cite the above experimental papers and either express optimism that the approach can surpass classical radar (given enough R&D) or skepticism about the technical hurdles. In summary, the literature on quantum radar spans groundbreaking theory (Lloyd 2008), proof-of-concept lab experiments (Waterloo 2019, Austria 2020), and strategic analysis pieces, each contributing to understanding how and whether quantum radar will ultimately fulfill its promise.
Use Cases and Applications
Military and Defense
The most headline-grabbing application of quantum radar is undoubtedly in the military domain – particularly for counter-stealth and advanced threat detection. Modern stealth aircraft like the F-35 or B-2 bomber are designed to evade classical radars by having a minuscule radar cross-section, reflecting away or absorbing much of the incident radar energy. A sufficiently sensitive quantum radar could flip this script. Because it can separate a target’s weak echo from overwhelming background noise, a quantum radar theoretically might detect even a “nearly invisible” stealth plane. Media outlets have speculated that quantum radar could render stealth technology obsolete by picking up the tiny returns that classical systems miss. In practical terms, a quantum radar scanning the skies could notice the quantum-correlated photons returning from a stealth jet and distinguish them from the ambient thermal photons, effectively spotting a needle of a signal in a haystack of noise. This capability is why China’s reported quantum radar tests – claiming detection of targets at 100 km – caused a stir, with some dubbing it a potential “stealth buster.” If an operational quantum radar could indeed track fighter jets or stealth bombers at long range, it would be a game-changer for air defense.
Beyond stealth aircraft, quantum radar could enhance detection across a range of military targets. For instance, it could improve tracking of ballistic missiles and warheads. Intercontinental ballistic missiles (ICBMs) and their warheads travel through space and can release decoys to confuse conventional radars. A quantum radar’s sensitivity and ability to discern real objects from fake (via the quantum signal’s behavior) might help missile defense systems discriminate a genuine warhead from inflatable or metallic decoy balloons during the mid-course phase of flight. The entangled photons’ interaction with a target could even give information on the target’s composition, potentially indicating whether it’s a heavy nuclear warhead or a lightweight decoy. This kind of detailed insight is extremely valuable for strategic defense. Another military use case is submarine detection – while radar is not used underwater, quantum sensing principles might be applied in other spectra (like magnetic sensing or optical ranging through water) to detect submerged vessels. It’s conceivable that a “quantum radar” in the broad sense, perhaps using entangled sonar signals or magnetic field quantum sensors, could one day help reveal stealth submarines that currently elude detection. Similarly, low-observable hypersonic missiles or high-speed drones (which may have small radar signatures and travel in cluttered environments) could be more reliably tracked. China’s military researchers have suggested installing quantum radars on near-space platforms (high-altitude balloons or UAVs) to watch for incoming missiles or even to monitor objects in low Earth orbit. In one concept, a stratospheric airship with a quantum radar could form a persistent early-warning node, spotting ballistic missiles as they boost toward space or surveilling stealth aircraft from above. In short, the primary military applications driving quantum radar R&D are those where classical radars struggle: detecting low-observable targets (stealth aircraft, stealthy cruise missiles), sorting out real threats from decoys, and operating in heavy jamming or clutter. If quantum radar lives up to its billing, it could significantly erode the advantages of today’s stealth and electronic warfare tactics.
Civilian, Scientific, and Other Applications
While defense gets the spotlight, quantum radar (and quantum illumination techniques) could have a variety of non-military applications as well. One oft-mentioned use is in air traffic control and aviation safety. A quantum radar system could potentially monitor civilian airspace with much lower power emissions, which means less interference with other systems and reduced radiation exposure. It might detect small objects like hobby drones or birds near airports that conventional radars have trouble picking out from ground clutter. Because a quantum radar can work in very noisy conditions, it could be useful in busy urban environments where there’s a lot of electromagnetic noise or multipath reflections. Imagine an air traffic control radar that can see a small quadcopter hovering near a runway despite the radar confusion caused by buildings, weather, and other aircraft – quantum-enhanced sensing might help achieve that level of fidelity.
In the realm of space exploration and remote sensing, quantum radar concepts could be used to probe environments that are challenging for classical sensors. For example, a quantum radar on a spacecraft might be able to detect objects in the asteroid belt or map planetary surfaces using very weak signals that blend into ambient cosmic noise for normal radar. Since quantum illumination works even with a low signal-to-noise ratio, a rover on Mars or a moon lander could use a quantum lidar/radar hybrid to see through dust storms or scan shadowed craters with minimal power. Another intriguing idea is using quantum radar principles for space situational awareness – tracking satellites and space debris. A network of quantum radars on Earth or in orbit might one day monitor even “stealth” satellites (if such become a reality) or debris fragments, by using correlations to filter out the noisy background of space. This is similar to the military space surveillance idea, but with a civilian twist for space traffic management.
Perhaps the most promising non-military applications are in imaging and sensing in challenging environments. Quantum illumination has been proposed for medical imaging and security scanning, where you want to detect objects with the least possible radiation. In medical diagnostics, for instance, one could envision a quantum radar-like device that uses entangled microwaves or terahertz waves to scan for tumors or other anomalies in tissue. Because the quantum approach could achieve the same imaging result with far fewer photons than a classical scanner, it might produce less tissue heating or damage. In fact, the quantum radar prototype in Austria was noted to have potential uses in “ultra-low power biomedical imaging.” It could operate like a very sensitive MRI or X-ray alternative, detecting subtle contrasts in tissue by bouncing weak microwaves and using quantum correlation to extract a clear signal. Similarly, in airport security scanners (the millimeter-wave scanners used to detect concealed weapons on passengers), quantum enhancements could reduce the power needed or improve the detection of low-reflectivity materials (like certain plastics or ceramics). Because the system can better distinguish signal from noise, it might catch items that today’s scanners miss or eliminate false alarms, all while using a gentler radiation dose.
Other scenarios include seeing through fog, smoke, or murky water. Firefighters could use a quantum radar-based imager to see people through thick smoke in a burning building, where classical infrared cameras are overwhelmed by hot smoke and classical radar doesn’t have the resolution. Quantum LiDAR (laser radar) could help autonomous cars navigate in bad weather by better distinguishing obstacles through heavy rain or fog. Oceanographers might use quantum entangled light to improve underwater imaging, since water is a very noisy and absorptive medium for sonar and light – time-correlated photon techniques could enhance seeing through turbulent water or locating objects on the murky seabed.
It’s important to note that many of these non-military applications are still speculative, and they face many of the same technical challenges as the military ones (noise, range, etc.). But the overarching theme is that any scenario that involves detecting weak signals in heavy noise or loss could benefit from quantum radar technology. This spans a surprisingly broad range: from tracking an aircraft with a tiny radar return, to distinguishing a tumor in a human body, to mapping a hidden room behind a wall (quantum radar has even been fancied for some forms of through-wall imaging for rescue or law enforcement). Each of these use cases would require tailoring the quantum radar’s frequency and design – e.g. using microwaves for long-range air surveillance, terahertz waves for medical or security scanning, optical photons for fine imaging – but the underlying quantum principle of entangled or correlated detection would be the common thread providing an edge over classical methods.
Current Status of Quantum Radar Development
So, where do we stand today with quantum radar? In short, it’s a technology in the proof-of-concept stage, with a few laboratory prototypes demonstrated, but far from operational deployment in the field. All existing quantum radar experiments have been at relatively short ranges – on the order of meters, not kilometers. In fact, current experimental designs are limited to very short distances (one recent review noted on the order of 1 meter range so far). The successes to date, like the Canadian and Austrian prototypes, have been in controlled environments (often cryogenic labs) showing that a quantum advantage in detection is real under those conditions. For example, the Austrian team’s microwave quantum radar detected a small object in a high-noise thermal environment, but this was essentially across a lab bench, not across a landscape. The Canadian quantum radar experiment similarly was a tabletop demonstration, albeit one that clearly validated the theory. These are important milestones, but a long way from a system that could, say, patrol the skies.
There are a few known prototype devices and ongoing projects internationally. China often claims to be leading in this area: in 2016, CETC announced it had built the “world’s first quantum radar” and later said it achieved detection at 100 km (62 miles) under test conditions. However, these claims have not been independently verified and are met with some skepticism. Chinese researchers did demonstrate a form of quantum ranging in the lab, but many Western scientists believe that scaling it to 100 km with useful sensitivity is extremely challenging with today’s technology. It’s possible China has a head start in engineering a quantum radar testbed (perhaps using a high-altitude platform to reduce background noise), but publicly available data is scarce. Aside from China, Canada’s DRDC in partnership with university labs has been active in quantum radar research (one of the few actual prototypes came from this collaboration, as noted). In Europe, there’s interest via the EU’s quantum initiatives – for instance, the UK’s Defence Science and Technology Laboratory and partners like Fraunhofer have looked into feasibility studies. The U.S. military has so far taken a more cautious approach: agencies like DARPA and IARPA are funding quantum sensing broadly (focusing on things like quantum clocks, magnetometers, etc.), and while they are certainly aware of quantum radar, the official stance (reinforced by the 2020 Lincoln Lab study) is that it’s not yet a practical near-term solution for long-range detection. That said, companies such as Raytheon and BAE Systems have disclosed internal research into quantum-enhanced radar techniques, likely to ensure they are not caught flat-footed if the technology advances suddenly. Raytheon, for example, has an “Intelligence & Space” segment study on quantum radars to improve radar performance via entanglement. BAE Systems has worked with DARPA on quantum sensing contracts that, while not explicitly “quantum radar,” could feed into related receiver technologies.
Major players in the field right now include government research labs (like China’s 14th Institute of CETC, Canada’s DRDC, and various U.S. Air Force or Navy research arms monitoring the tech), academic groups (University of Waterloo and University of Calgary in Canada, IST Austria, University of York in the UK, MIT in the U.S., University of Science and Technology of China, etc.), and some private sector efforts primarily tied to defense contractors (Lockheed Martin had early patents; Raytheon and Northrop Grumman have interest in next-gen radar; and startups or smaller companies may spin off if a breakthrough occurs). It’s worth noting that the National Quantum Initiative and similar programs abroad mean there is significant funding available for quantum sensing research, so new prototypes or incremental advances are likely in the coming years.
Despite the progress, formidable technological barriers remain before quantum radar can be truly practical. One big challenge is decoherence and noise – the very environments we want quantum radar to work in (lots of noise, long distances) are the ones that destroy fragile quantum states. For instance, maintaining entanglement or useful quantum correlations over a long distance requires either a way to protect photons from environmental interference or a method to store the idler photons until the signal returns. Storing the idler is problematic; it essentially means having a quantum memory that can hold a photon state for the round-trip time of the radar pulse. For a target tens of kilometers away, that might be hundreds of microseconds or more, which is an eternity for quantum coherence. Using a simple delay line (like a long optical fiber spool) to delay the idler leads to significant degradation of the quantum state, limiting the effective range. Studies indicate that using a standard fiber delay might cap a quantum radar’s range at around 10–11 km before the quantum advantage washes out. Beyond that, you’d need quantum memories or repeater-like mechanisms, which are an active research topic but far from deployment.
Another issue is the need for cryogenics and specialized hardware. Many quantum radar concepts rely on superconducting devices (like parametric amplifiers or single-photon detectors) operating at millikelvin temperatures to generate or measure entangled microwaves. The Austrian experiment, for example, entangled microwaves using a device cooled to a few thousandths of a degree above absolute zero. This is clearly not ideal for a field-deployed radar on a ship or an aircraft, although future engineering might produce portable cryocoolers or even room-temperature quantum sources using different physics. On top of that, quantum radars currently only use a single transmit/receive channel in experiments – unlike classical radars that have arrays of antenna elements for directional beamforming, polarization diversity, etc. Extending quantum entanglement to multiple spatial modes (or many frequencies at once) is extremely complex. Right now, designs consider one polarization, one direction, one range bin at a time. A practical radar would need to scan or cover many directions and ranges, which means scaling up the quantum system by orders of magnitude or finding clever multiplexing strategies.
Perhaps the biggest limitation is range and power. Classical radar can simply crank up the transmit power or use large antennas to see farther; quantum radar cannot easily brute-force its way to long range because the fragile quantum states don’t survive a high-power long-distance journey. Paradoxically, quantum radar excels at low-power situations – it’s almost a “low-SNR specialist.” But if you try to use it in high-SNR regimes, classical radar already does fine and there’s no advantage to gain. Some experts argue that for long-range detection (tens to hundreds of kilometers), a well-designed classical radar (using techniques like pulse compression, coherent integration, MIMO arrays, etc.) can perform as well as or better than a quantum radar, without the hassle of cryogenics and entanglement. In fact, a 2020 MIT Lincoln Lab feasibility study flatly stated “Quantum radar does not have the potential for long range standoff sensing (>10 km) at radio frequencies” with current technology. They cited not only hardware limits but also the problem that to detect a target at long range with quantum illumination, you might need to integrate (collect signals) for an impractically long time – one analysis suggested up to years of data collection for a single pulse’s return in certain scenarios. Clearly, no one can wait years to detect a speeding aircraft! The U.S. Defense Science Board was similarly unconvinced, concluding in 2019 that quantum radar “will not provide [an] upgraded capability” to the Department of Defense in any foreseeable timeframe.
All that said, the current status is not static. Researchers are actively looking for solutions to these challenges. For example, there’s work on better quantum sources and detectors that operate at higher temperatures, on quantum memory devices that might store microwave photons (using exotic approaches like spin ensembles or mechanical resonators), and on integrating quantum radar concepts with classical radar infrastructure to get the best of both. There’s also exploration of alternative quantum radar architectures that might be more practical. One idea is using pre-squeezed or pre-entangled states in a clever way that doesn’t require storing the idler for as long, or using entanglement swapping so that the idler and signal are compared through an intermediary step. Another approach is to leverage optical frequencies (where generating entanglement and detecting single photons can be done at room temp with existing tech) and only convert to microwave at the last moment for transmission – this was the 2015 proposal and remains a promising route if conversion efficiency improves. On the hardware side, companies are miniaturizing quantum photonic components. There’s talk that the first portable quantum radars might actually operate at shorter range but find niche uses like covert perimeter surveillance or near-distance security scanning, as hinted by some reviews.
In summary, as of today quantum radar is a technology in an embryonic stage: proven to work in principle on the lab scale, but facing a steep climb to become broadly useful. No military has an operational quantum radar yet (despite any unverified claims). The major players are continuing research, with China seemingly pushing hardest for a strategic system and others content to support lower-level R&D for now. The coming years will likely see incremental improvements – e.g., a prototype that works over, say, 100 meters, then 1 km, perhaps in a closed environment – rather than a sudden deployment of a stealth-detecting super-radar. But given the pace of quantum tech development, surprises can happen. The field remains a vibrant intersection of cutting-edge physics and engineering, with the current status best described as “promising, but not yet game-ready.”
Comparison with Classical Radar
How exactly does quantum radar stack up against classical radar, and in what ways is it better or worse? It turns out that quantum radar offers some clear theoretical advantages, but also comes with significant limitations, especially at present. Here’s a breakdown of key points of comparison:
Detection Sensitivity: In scenarios with extremely low signal returns and high background noise, quantum radar can outperform classical radar. For instance, at equal low power, a quantum radar might detect a target where a classical radar is overwhelmed by noise. Experiments have shown a quantum-enhanced radar detecting objects when the signal level is around the single-photon level, vastly outdoing a classical analog in those conditions. This means if you’re limited in how much signal you can send (for stealth or safety reasons), quantum methods can give you a higher probability of detection. Classical radars, however, can compensate by increasing power or using techniques like integration (averaging multiple pulses) – but there’s a point where simply more power isn’t an option (either due to regulations, power availability, or the target’s stealth absorption). Quantum radar shines in that ultra-low-SNR regime.
Range: Classical radar has the clear upper hand in range today. Modern military radars can track large aircraft hundreds of kilometers away by using high-power transmitters and large antenna arrays. Quantum radar prototypes, by contrast, have only achieved meters to maybe a few kilometers (if we are generous with unverified reports) of range. The fundamental range of a radar is related to transmitted power, antenna gain, and sensitivity. Quantum radar, paradoxically, doesn’t aim to increase raw power or gain; instead it reduces the required SNR for detection. In theory, you could get similar range with much lower power if quantum correlations hold. But practically, maintaining those correlations over long distance is extraordinarily hard. As noted, a U.S. study asserted quantum radar won’t be viable for >10 km standoff sensing with current tech. So classical radar wins in range until some breakthrough allows quantum radar to scale.
Resolution and Information: Quantum radar might offer some improvements in the quality of information one can get from a target. In conventional radar, resolution (in range, angle, velocity) depends on factors like bandwidth, antenna aperture, and waveform processing. Quantum radar doesn’t magically increase bandwidth or antenna size, but some research suggests entangled photons could achieve better resolution with the same resources. A 2019 theoretical protocol showed that entanglement could localize a target in 3D space with quadratically better precision in each direction compared to unentangled photons. Additionally, as the Chinese researchers pointed out, a quantum radar could potentially glean target characteristics (like material composition or exact shape) by analyzing how the quantum state of photons is changed by interaction with the target. For example, the phase or polarization changes in entangled photons might carry a fingerprint of the target that classical radars can’t easily detect. However, this is largely theoretical at this point; classical radars combined with advanced signal processing (or multi-static setups) can also extract a surprising amount of info about a target. So the jury is out on whether quantum radar will materially improve resolution and target characterization in practice or if it’s just another way to achieve what could be done classically.
Stealth Detection: This is where quantum radar is touted to have a major edge. Classical radar struggles with stealthy objects that are designed to reflect very little energy back. You can try lower-frequency radars (stealth aircraft often have a bigger signature at lower frequencies like UHF/VHF, but lower frequency means worse resolution), or multi-static radar nets (catch a stealth plane from multiple angles), or non-radar methods (infrared sensors, etc.). Quantum radar provides a new avenue: it looks for correlated returns rather than sheer signal strength. A stealth plane might reduce the power of the return by 1000x, but as long as even a few entangled photons come back, the quantum radar knows exactly what to look for (it has the idler twin of those photons). Thus even a tiny number of return photons, hidden in thermal noise, could be picked out. In concept, this makes a stealth aircraft much more detectable to a quantum radar than to a classical one. In effect, quantum radar could negate the main advantage of stealth (low observable by radar) by making the noise itself the reference point – if something is there, the noise isn’t truly random anymore from the radar’s perspective. That said, until a quantum radar actually tracks a stealth aircraft in the field, this remains an anticipated advantage. There is also the counterpoint that advanced classical radar techniques (like passive radar using reflections of civilian signals, or bi-static configurations) might also catch stealth objects, so stealth is a moving target in more ways than one.
Resistance to Jamming and Deception: Quantum radar has a clear theoretical advantage in resilience to electronic warfare tactics. Traditional radars can be jammed by flooding them with noise or spoofed by clever repeaters that send false echoes. Since a classical radar just sees incoming waves, an enemy jammer can send strong signals to confuse it. But a quantum radar’s receiver is looking for specific quantum correlations that come only from its own transmitted photons. If an adversary floods the radar with regular (un-entangled) radio noise, the quantum radar can effectively ignore it because those signals won’t have the right correlation with the stored idler photons. It’s as if the radar is “listening” for a secret password in the returning signal – noise and fake signals don’t know the password. Any attempt to mimic the entangled signal would require the jammer to somehow capture the quantum state or replicate it, which quantum mechanics forbids (cloning of an unknown quantum state is not possible). As a result, attempts to spoof a quantum radar would be easily noticed, since tampering with the signal breaks the delicate quantum correlations. This implies a quantum radar could operate in heavy jamming environments far better than classical radars, maintaining reliable detection where classical systems might be blinded or tricked. On the flip side, classical radars have developed other anti-jamming techniques (frequency hopping, adaptive filtering, etc.) but they can still be overwhelmed by sufficiently powerful jammers or fooled by sophisticated decoys. Quantum radar, by design, carries an inherent authentication of its signal via entanglement, which is a powerful defense against spoofing.
Complexity and Cost: Currently, quantum radars are extraordinarily complex compared to classical radars. A classical radar is a well-understood engineering system: transmitter, antenna, receiver, signal processor. A quantum radar requires all that plus a quantum source (entangled photon generator), quantum-grade receivers (sensitive single-photon detectors or parametric amplifiers), possibly cryogenic cooling, and careful timing to correlate signals. This means, at least in the near term, a quantum radar would be much more expensive and maintenance-heavy than an equivalent classical radar. Even if it provides better performance in some niche, users will weigh whether the complexity is justified. Over time, as quantum tech matures, this may change – what is exotic today (like a stable entangled photon source) could become as routine as a laser diode. Classical radars will also continue to get cheaper and better with improved electronics. Right now, building a quantum radar is a research project costing significant money; building a classical radar is something that can be done off commercial components relatively affordably. So in practical comparison, classical radars win hands-down on cost, robustness, and readiness. Quantum radars, if they become deployable, might initially be reserved for special roles (where their unique advantages outweigh their cost/complexity).
To put it succinctly: quantum radar isn’t about replacing classical radar across the board. Instead, it’s expected to complement or surpass classical radar in specific challenging scenarios, like detecting extremely faint or stealthy targets or operating in extreme noise/jamming. In more ordinary tasks (like tracking non-stealthy aircraft in clear weather at medium range), classical radar is likely to remain more efficient and capable for a long time. There is some healthy skepticism in the radar expert community; some liken quantum radar today to the early days of aviation – promising but primitive. One radar engineer wryly noted that unlike the Wright brothers (who had birds as proof that flight was possible), quantum radar pioneers don’t yet have an existence proof of long-range quantum detection in nature. Nonetheless, the potential advantages (stealth detection and jam-resistance in particular) make quantum radar a tantalizing goal, and ongoing comparisons with the best classical techniques will continue to inform whether it can truly outperform them in the real world.
Security, Ethical, and Geopolitical Implications
If quantum radar technology progresses to practical use, it could have far-reaching implications for global security and military strategy. The most immediate impact would be on the balance of power in stealth warfare. Since the late 20th century, stealth aircraft and vessels have given militaries (notably the United States) a significant edge, allowing them to conduct operations with reduced risk of detection. Quantum radar threatens to upend that advantage. A world where quantum radars are widely deployed is a world where stealth might no longer mean invisible. Militaries would have to assume that their once-low-observable bombers, fighters, or drones can be seen by adversaries, at least under certain conditions. This could diminish the deterrent and first-strike value of stealth forces. For example, a fleet of stealth bombers as a strategic deterrent works only if the enemy can’t track them; if a rival can track those bombers reliably with quantum radar, the stealth force might be neutralized or at least loses its shock-and-awe advantage. This shift could encourage development of countermeasures – perhaps counter-quantum techniques (like creating deliberate quantum noise to mask returns or new stealth materials that absorb quantum-correlated photons in a tricky way). It might also shift focus back to other delivery systems, like missiles, if crewed stealth aircraft become more vulnerable.
The prospect of quantum radar is already fueling a subtle arms race in sensing. China’s vigorous investment and bold claims have likely spurred other nations to invest more in their own quantum radar research or at least in counter-stealth alternatives. If one country were to achieve a functional long-range quantum radar first, it would have a window of opportunity to negate the stealth assets of its rivals, potentially upsetting strategic stability. This is especially pertinent to the US-China scenario: The U.S. has a large stealth aircraft arsenal; China developing quantum radar could erode U.S. superiority in a Taiwan Strait or South China Sea contingency by making U.S. jets and bombers easier to target. That in turn might push the U.S. to accelerate its quantum sensing projects or find new ways to hide (like electronic decoys, or investing more in hypersonic missiles and submarines that don’t rely on stealth in the same way). In effect, quantum radar could intensify the military technology race, as each advance in detection (quantum radar) is met with a counter (maybe “quantum stealth” or other tactics). We’ve seen similar cycles historically: for instance, radar led to radar jammers and stealth planes; quantum radar would be a new round in this cat-and-mouse dynamic.
Beyond stealth, quantum radar could undermine elements of nuclear deterrence and strategic stability. One pillar of nuclear deterrence is the second-strike capability – the assurance that even if a country is attacked, it can retaliate, often thanks to stealthy platforms like nuclear submarines or elusive strategic bombers. If quantum sensors make the oceans transparent to detect submarines (through quantum magnetometers or gravimeters) and make the skies transparent to stealth bombers via quantum radar, a nation might fear its second-strike assets are vulnerable. This could lead to destabilizing behavior, such as keeping nuclear forces on higher alert or investing in even riskier technologies. On the other hand, if quantum radar is deployed defensively (e.g., as an early missile warning system or to better track potentially hostile aircraft entering one’s airspace), it might enhance stability by reducing false alarms and miscalculations. For instance, a high-fidelity detection system could prevent accidental escalation by clearly distinguishing a flock of birds or a weather balloon from an incoming stealth cruise missile – something that today’s radar occasionally has trouble with.
Ethically, the development of quantum radar raises some interesting questions. If it indeed nullifies stealth, does it make warfare more or less ethical? One could argue that it might reduce surprise attacks on civilian targets (since attacking forces can be detected earlier), potentially saving lives through better early warnings. It could also discourage nations from investing huge sums in stealth strike weapons and maybe shift focus to defense. Conversely, if one side believes it has an uncounterable quantum radar, it might be emboldened to undertake offensive operations, thinking the enemy cannot strike back invisibly. There’s also an argument that quantum radar, as a sensing technology, could stabilize situations by making military postures more transparent – similar to how satellite surveillance, though initially military, has contributed to transparency and trust (e.g., arms control verification). However, transparency cuts both ways; it can also make it harder for countries to hide defensive deployments, which they might feel are necessary.
Another aspect is the geopolitical race in quantum technology more broadly. Quantum radar is one piece of a larger puzzle including quantum computing, communication, etc. Countries that lead in quantum radar also signal overall quantum tech leadership. China’s hefty funding (the Hefei lab, etc.) and their public statements on quantum radar indicate they want to claim the mantle of being first with this capability. The U.S. and Europe, while perhaps quieter about quantum radar specifically, are certainly aware that falling behind in quantum sensing could have national security implications. We’ve already seen high-profile “quantum Sputnik moments” like China’s quantum-encrypted satellite; a successful demonstration of a quantum radar detecting a stealth fighter in a test would likewise reverberate globally.
There is also an information security concern: if quantum radar becomes reality, stealth technology (which many nations have invested in) suddenly loses value. Countries might double down on other means of achieving surprise or protection, some of which could be more dangerous (like deploying more decoys that could be mistaken for real warheads, or adopting launch-on-warning postures for nuclear forces out of fear their assets will be tracked and destroyed preemptively). Ethicists might worry that by undermining stealth second-strike platforms (like stealthy nuclear cruise missiles or bombers), quantum radar could inadvertently lower the threshold for a nuclear first strike by an adversary (since the adversary might believe it can knock out all detectable assets, or conversely the side with stealth might use them earlier before quantum radar proliferates).
On the positive side, quantum radar and related quantum sensors could enhance security beyond the battlefield. For example, in counter-terrorism or law enforcement, a quantum radar could better detect smuggled weapons or stealthy drones used by non-state actors. It might guard critical infrastructure by seeing through cloaking measures or finding hidden surveillance devices (imagine sweeping an area with entangled photons to detect a tiny spy drone or a camouflaged object). Used in this way, it could protect civilian lives and reduce the advantage bad actors might get from stealth or simply darkness and noise.
In any case, the advent of quantum radar will force strategic recalculations. Nations are already developing response plans: some may invest in quantum countermeasures, others in their own quantum radars to avoid a capability gap. We might see international discussions on quantum radar if it comes close to deployment – perhaps even attempts at arms control for certain quantum sensing capabilities (though that seems far off and hard to enforce). The ethical implications will largely hinge on how the technology is used: purely as a defensive sensor (arguably stabilizing), or as part of an offensive strategy to nullify adversary defenses (potentially destabilizing if one side gains a temporary edge). As with most dual-use technologies, global stability will depend on transparency, dialogue, and possibly agreements that manage the impact of seeing “the invisible.” In the meantime, the mere possibility of quantum radar is already influencing military planners and defense budgets, as the world’s powers prepare for a future where quantum sensors could rewrite the rules of detection and deterrence.
AI and Quantum Radar: A Powerful Combination
Marrying artificial intelligence with quantum radar could significantly amplify the capabilities of this nascent technology. Quantum radar systems will generate complex datasets – correlations between signal and idler photons, statistical patterns in noisy returns, and so on – which are not as straightforward to interpret as classical radar blips. This is where AI-driven signal processing comes in. Machine learning algorithms, especially deep learning neural networks, excel at finding patterns in data and making sense of noisy inputs. In a quantum radar, an AI could be trained to recognize the subtle signatures of a target’s presence in the quantum correlation data. For example, instead of using a simple threshold detector, one could employ a trained neural network to analyze the joint measurement outcomes from thousands of signal-idler photon pairs and decide if a target is present with higher confidence. Such an AI system might learn to filter out sporadic environmental noise or instrument artifacts that a traditional algorithm might mistake for a target. Researchers are already using deep learning for classical radar tasks like clutter suppression and jamming recognition, and these techniques can be extended to quantum radar data, which also faces clutter (from thermal noise) and potential jamming attempts.
One promising avenue is using AI for noise reduction in quantum radar. Quantum states are susceptible to environmental disturbances, and a lot of irrelevant signals (background thermal photons, cosmic rays, etc.) can hit the receiver. An AI could learn the statistical profile of genuine entangled returns versus spurious noise and thereby improve the effective sensitivity of the radar. In essence, it could serve as an intelligent filter, boosting the signal-to-noise ratio beyond what the quantum correlations alone provide. Additionally, AI-based algorithms could perform pattern recognition on the quantum radar’s output. Suppose a quantum radar is scanning and picking up tiny returns; an AI system might be better at recognizing the pattern over time that indicates a moving aircraft, as opposed to random fluctuations. This is analogous to how AI is used in astronomy to spot exoplanets or pulsars in noisy data – except here it’s spotting, say, a stealth drone’s signature in quantum radar readings.
Another area where AI can contribute is real-time adaptive control of the quantum radar. Quantum systems often have many tunable parameters (for instance, the degree of squeezing, the frequency of operation, the modulation of pulses, etc.). A reinforcement learning agent – a type of AI that learns by trial and error – could dynamically adjust these parameters to optimize detection probability as conditions change. If the environment’s noise level rises (maybe due to the sun heating the atmosphere or an adversary trying to jam with thermal noise), the AI could adjust the radar’s measurement settings or the way it post-processes the data to maintain performance. Essentially, AI could give the quantum radar a form of “autopilot” or self-optimization, something that would be invaluable in the field where conditions are unpredictable.
We should also consider the synergy of quantum computing and AI in the context of radar. In the long run, one could imagine using a quantum computer to directly process quantum radar returns – a concept sometimes termed quantum machine learning for sensing. That’s a bit futuristic, but there are already discussions about using quantum algorithms to enhance classical radar signal processing. More practically in the near term, classical AI algorithms can be employed to assist quantum radar prototypes. For instance, a support vector machine or neural net could be trained on simulation data of quantum radar returns (with and without targets) to create a very efficient detector that outperforms classical statistical tests. Militaries are likely investigating such combinations quietly; any advanced radar coming out today, quantum or not, is usually paired with sophisticated software for target discrimination.
Imagine a scenario: a quantum radar picks up a possible stealth target. Instead of a human operator squinting at a screen of noise, an AI agent has already cross-correlated the quantum data, identified a pattern matching a high-speed aircraft, filtered out all known false-positive patterns (like birds or weather anomalies), and provides a confidence score that “yes, there’s a stealth UAV at these coordinates moving north-east.” This reduces the chance of error and speeds up response decisions. AI could also coordinate multiple quantum radar sensors – if you deploy a network of quantum radars (perhaps smaller units), an AI system could fuse their data, much like sensor fusion in classical systems, to build a composite picture that’s more accurate than any single unit’s view.
There are some existing initiatives that hint at AI-assisted quantum sensing. While not specific to radar, the U.S. DARPA’s programs on Robust Quantum Sensors implicitly consider that any deployed quantum sensor will need smart error correction and interpretation (roles suited for AI). It’s reasonable to expect that by the time quantum radar is mature enough to test outdoors, machine learning will be integral to its signal processing chain. The Chinese research community, which is strong in both quantum tech and AI, has likely considered pairing them as well – perhaps using AI to overcome some of the noise issues in their prototypes, although concrete details aren’t public.
In summary, artificial intelligence acts as a force multiplier for quantum radar. It can mitigate some of the weaknesses of quantum radar (like sensitivity to noise and huge data volumes) and enhance its strengths (extracting maximum information from minimal signals). An AI-empowered quantum radar system could autonomously adjust to counter enemy jamming, discern real targets from ghost signals with higher accuracy, and possibly even identify the type of object detected by learning its “quantum silhouette.” This combination of AI and quantum sensing could yield an ultra-smart radar that is both quantum-aware and context-aware. It’s a powerful pairing: quantum radar provides the eyes that can see what was once invisible, and AI provides the brain to understand what those new eyes are seeing.
Future Outlook
Looking ahead 10 to 20 years, will quantum radar live up to its hype as a disruptive force, or will it remain a niche scientific curiosity? The likely outcome lies somewhere in between, but leaning toward gradual, niche adoption unless major breakthroughs occur. In the next decade, we can expect quantum radar research to continue aggressively, with improvements in range and reliability coming step by step. We may see intermediate milestones like a quantum radar demonstrator that works at, say, 1 km range against a specially instrumented target, or a field trial of a short-range quantum radar for perimeter security or naval use. These incremental successes will help convince stakeholders that the concept is viable, while also highlighting what still needs work.
One possible development in the near future is the emergence of special-purpose quantum radars. For instance, perhaps a quantum radar specifically designed for short-range high-clutter environments (like an urban battlefield or a ship’s close-in surveillance) becomes practical. Such a radar might only have a range of a few kilometers, but in that domain it could outperform conventional sensors by spotting very low-signature threats (like a stealthy mini-drone or a periscope of a mini-submarine in choppy waters) that confuse classical radars. Militaries might deploy these as supplements to traditional radar – essentially another sensor in the toolkit used when needed. Similarly, there could be quantum radars for ground-penetrating radar (to find hidden tunnels or IEDs with less false clutter) or for through-wall imaging in special forces operations, where again the range is limited but the environment is noisy and hard for classical systems.
In parallel, we anticipate significant technological advances that will push quantum radar forward. One such area is quantum computing and error correction, which, while usually discussed in the context of computation, could translate to sensing. Techniques from quantum error correction might be employed to make entangled states more robust against loss – essentially creating “error-corrected entanglement” that survives longer, extending radar range. Another area is the development of better entangled photon sources and photonic integration. If researchers can integrate entangled photon generators and detectors onto semiconductor chips (much like today’s photonic integrated circuits for telecom), quantum radar hardware could shrink in size and complexity. There’s already work in creating entangled photon pairs on chips using nonlinear optical resonators. In 10-20 years, we might have a compact module that produces entangled microwave photons at GHz rates, no bulky lab equipment required.
Improved quantum memories or delay techniques will be critical if quantum radar is to go long-range. We might see novel quantum storage approaches – for example, storing the idler photon’s information in a spin ensemble or converting the microwave photon to an optical one and storing it in a doped crystal (quantum memories used in quantum communications research) – that allow a longer delay without losing the quantum correlation. If one could hold an idler for even a few milliseconds without decoherence, that corresponds to a one-way range of ~300 km (since light goes 300 km in 1 ms). That’s very ambitious, but even a 100 microsecond storage (15 km one-way) would be a game changer for radar range. Hybrid systems might arise: maybe a quantum radar that uses entanglement for short-range detection and switches to classical mode for long range, essentially a multi-mode radar that chooses the best method for the scenario.
Another trajectory is using different quantum resources beyond entanglement. There’s theoretical work on quantum sensing that doesn’t rely on entanglement but other phenomena like quantum squeezing or even exotic ideas like using time-frequency entanglement (where photons are entangled across frequency bands). It could turn out that a highly squeezed state of microwaves (which is easier to produce than entangled pairs) offers a substantial radar advantage by reducing noise in the measurement of the return signal’s phase or amplitude. In such a case, future “quantum radars” might be more like extremely optimized classical radars that operate at the quantum noise limit, using states of light that blur the line between classical and quantum (a squeezed state is technically a non-classical state). This might be easier to implement than a full entangled-photon quantum illumination scheme and could bridge the gap sooner.
In terms of being disruptive, quantum radar could become a dominant anti-stealth technology by the 2040s if current progress continues and no insurmountable roadblocks emerge. By then, perhaps stealth aircraft designers will be grappling with the reality that their planes can be seen by quantum sensors. This could spur a shift in aerospace design – maybe focusing on speed and electronic countermeasures rather than geometric stealth, or integrating onboard quantum jammers to confuse quantum radars (if such a thing is possible). On the other hand, if quantum radar hits a wall (say, it can’t get beyond a few kilometers without losing too much advantage), it might remain a niche tool. It could end up like nuclear fusion in energy – always 20 years away. However, given that we have already seen functional prototypes, it feels more tangible than fusion.
One must also consider integration with other quantum technologies. Perhaps in 20 years, a quantum radar is just one component of a larger quantum sensing suite on a military platform. That suite might include quantum gravimeters (to detect underground structures), quantum magnetometers (for submarines), and quantum clocks (for precise navigation). If all these come together, the way warfare and surveillance is conducted would shift toward a more quantum-informed approach: stealth might be countered by sensors that observe not just electromagnetic reflections, but minute gravitational or magnetic anomalies – all requiring a rethink of how to hide and how to detect.
In the civilian world, if quantum radar becomes practical, it could quietly permeate technology. For example, an airport might have a quantum radar system to monitor the runway for FOD (Foreign Object Debris) – something classical radar can’t do well because a small bolt on the runway doesn’t reflect much. Or cars in 2040 might have quantum LIDAR options for the worst weather conditions, giving them an edge in safety during blizzards by seeing obstacles through the noise. These are speculative, but they indicate that if cost and size come down, quantum sensing could be as ubiquitous as GPS is today (GPS relies on atomic clocks – a quantum technology – which fifty years ago might have sounded too high-end to be in every phone).
For quantum radar to accelerate in viability, several research directions will be key: high-rate entangled photon generation (so the radar can get results faster and integrate results quickly), robust entanglement distribution (so that entangled photons survive the transmit and return path or can be effectively compared even with some loss), and interface between optical and microwave regimes (since many enabling technologies are easier in optics, making a good microwave-to-optical converter as proposed in some designs could leverage the best of both worlds). Progress in quantum computing could indirectly help by producing better qubits and control techniques that might be repurposed for sensing. Also, lessons from quantum communication (like quantum cryptography over satellites) could help in handling entangled photons over long distances, which is analogous in some ways to what quantum radar tries to do with a round trip.
In conclusion, the next 10–20 years of quantum radar will likely see it evolving from lab curiosity to at least a specialized operational tool. It may not replace your local weather radar or Air Force early-warning radar by 2040, but it could be sitting alongside them, doing things those radars can’t. If breakthroughs are achieved, especially in extending range, then quantum radar could indeed be disruptive, forcing a reimagining of stealth strategy and sensor warfare. If progress is slower, it will still enrich the field of sensing and find niche uses where its unique powers matter most. One thing is certain: the pursuit of quantum radar has already driven innovation in quantum optics and microwave technology, and that will continue to yield benefits, even if indirectly.