Quantum Repeaters: The Key to Long-Distance Quantum Comms
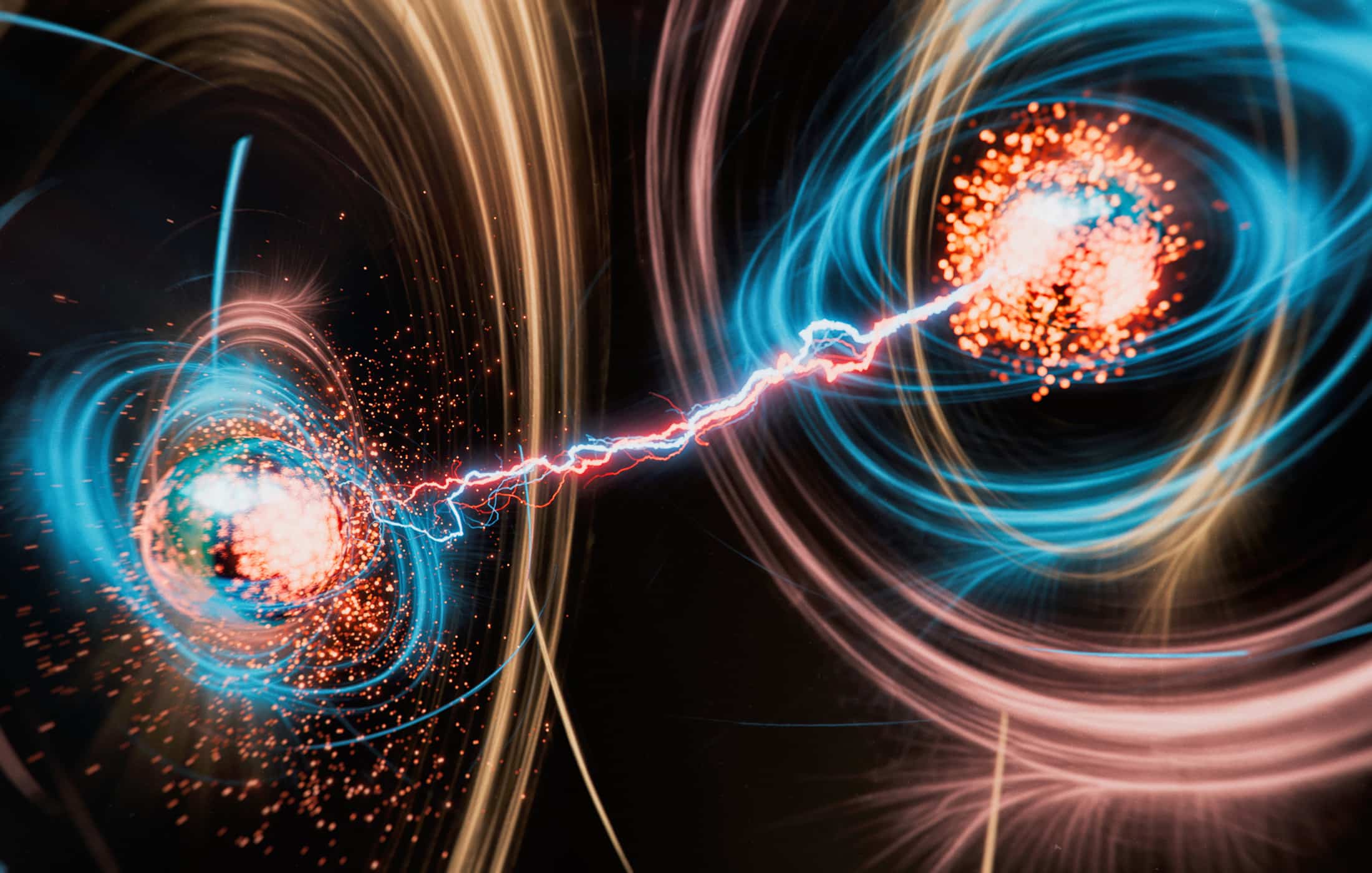
Table of Contents
Introduction to Quantum Repeaters
Quantum repeaters are specialized devices in quantum communication networks designed to extend the distance over which quantum information (qubits) can be sent without being lost or corrupted​. They tackle a fundamental challenge: photons carrying qubits tend to get absorbed or scatter as they travel through fiber or air, and quantum states can decohere (lose their quantum properties) due to environmental noise. In a direct fiber link, the signal from a single photon is quickly attenuated – for example, after only tens of kilometers, most photons are lost, and beyond a few hundred kilometers virtually none get through​. Moreover, qubits cannot be amplified like classical signals because any measurement or attempt to copy a quantum state will disturb it (as dictated by the Heisenberg uncertainty principle and the no-cloning theorem)​. This means the classical solution of using repeaters to boost signal strength fails for quantum data. A new approach is needed to send quantum information across continental distances.
Quantum repeaters provide that solution by leveraging entanglement and quantum memory instead of measurement and amplification. In essence, a quantum repeater breaks a long communication distance into shorter segments and creates entangled quantum states across each segment. Through a process called entanglement swapping, these segments can be joined, extending entanglement step-by-step over the full distance without ever measuring the intermediate qubits​. By teleporting quantum states from one segment to the next using entanglement, the fragile quantum information is relayed across the network without directly transmitting a single photon end-to-end​. Throughout this process, quantum memories at the repeater nodes temporarily store qubits, ensuring that entanglement can be established and synchronized across segments despite inevitable delays​. In short, quantum repeaters enable long-distance quantum communication by overcoming photon loss and decoherence, all while obeying quantum laws that forbid simple signal boosting​.
The fundamental principle behind a quantum repeater is “divide and conquer”: rather than send a delicate qubit directly over a lossy 1000 km fiber (almost certainly failing), we entangle shorter links (say 100 km each) and then connect them via entanglement swapping. Because each smaller link can succeed with manageable loss, and because the entanglement swapping procedure teleports the quantum state across the links, the end parties end up sharing a quantum state as if they were directly connected​​. Crucially, none of the intermediate nodes ever learn or copy the actual quantum data – they perform only quantum measurements (like Bell-state projections) that do not reveal the key or quantum state. This preserves the quantum coherence across the entire distance. In effect, a quantum repeater chain creates an end-to-end entangled pair between distant users, allowing them to perform quantum communication tasks (like establishing secret keys or teleporting quantum information) as if they had a direct line, even though physically the line is broken into segments. By addressing the problems of photon loss and decoherence in this quantum way, quantum repeaters make the vision of ultra-long-distance quantum communication – and ultimately a quantum internet – possible​.
Why Quantum Repeaters Are Critical for Quantum Networks
In today’s quantum communications, distance is a formidable enemy. Without repeaters, direct quantum links (for example, optical fibers carrying single photons) face an exponential drop-off in signal with distance​. Even under ideal conditions, quantum key distribution (QKD) – the best-known quantum communication protocol – is limited to on the order of a few hundred kilometers before the key rate plummets to unusable levels. Experimental QKD systems have stretched this as far as ~500 km using specialized techniques, but only with extremely low data rates​. This intrinsic limitation is known as the repeaterless bound – a fundamental rate-versus-distance limit for quantum signals. In practical terms, if you try to perform QKD between two cities 1000 km apart without quantum repeaters, you have essentially two options, both with drawbacks: (1) trust intermediate nodes to break the route into shorter hops (sacrificing end-to-end security), or (2) use quantum satellites to bridge the distance through space (which is expensive and only works under certain conditions like clear night skies). Neither is an ideal or scalable long-term solution for a global quantum network.
Quantum repeaters are critical for overcoming these limitations. They enable long-distance QKD with unconditional security by eliminating the need to trust intermediate parties. Without repeaters, early QKD networks (such as the 2,000 km Beijing–Shanghai fiber backbone in China) had to rely on dozens of “trusted nodes” that performed key hand-offs every 80–100 km​. This allowed quantum-encrypted links over long distances, but at the cost of breaking the quantum security chain at each node – each intermediate node could theoretically be a point of compromise. A true quantum repeater network, by contrast, would let Alice and Bob (the end users) share entanglement directly across the entire distance, so that only they possess the secret key, with no third party in the middle ever obtaining it. This is a game-changer for quantum key distribution. It means QKD can be extended from metropolitan scales to inter-city, inter-country, even intercontinental scales without loss of security. As one national lab put it, “Quantum key distribution is a promising technology that would rely on quantum repeaters… If we want QKD to be efficient and effective, we’ll need to spread the network over large distances”. In essence, quantum repeaters unlock the long-distance potential of quantum cryptography, enabling truly secure communications on a global scale – something impossible with direct links alone.
Another reason repeaters are critical is that quantum networks are not just for keys. Beyond QKD, a future quantum internet would let users transmit quantum states for various applications – e.g. connecting remote quantum computers or sensors. Here, classical repeaters are of no use because they would destroy the very quantum information we want to send. A quantum computer, for instance, can only be networked with another quantum processor via entanglement; measuring the qubits to send classical bits defeats the purpose. Quantum repeaters are the enabling technology for these networks, allowing entanglement to be distributed over long distances and thereby linking quantum devices. In fact, the only way to securely and remotely operate a quantum processor (say, a cloud quantum computer) is through a quantum link that preserves qubit states end-to-end​. By faithfully relaying quantum states, repeaters could someday connect distant quantum processors into a distributed quantum computer much as classical repeaters connect computers into the classical internet. They are, in effect, the backbone of the envisioned quantum internet, vital for both quantum cryptography and advanced applications like remote quantum computation and sensing.
It’s also important to note how different quantum repeaters are from classical repeaters. In classical networks, a repeater (or router/amplifier) simply reads the incoming bits and forwards a copy – a routine operation since copying classical data is trivial. In quantum mechanics, copying an unknown qubit is forbidden – the no-cloning theorem says you cannot make a perfect duplicate of an arbitrary quantum state​. Moreover, measuring a qubit to read it will typically destroy its encoded information. Therefore, a quantum repeater cannot “measure-and-resend” the signal as a classical repeater would​. This fundamental difference is why quantum repeaters must employ entanglement and quantum memories instead of amplification. They preserve quantum information without ever fully reading it, something no classical device can do. In summary, quantum repeaters are critical pieces of infrastructure because they overcome the distance barrier for quantum communication in a way that maintains the uniquely powerful security and integrity properties of quantum mechanics, which classical approaches would squander.
Key Technologies Behind Quantum Repeaters
Building a functional quantum repeater is one of the great technological challenges of quantum information science. It requires combining several cutting-edge quantum technologies. The core ingredients of a quantum repeater include entanglement swapping, quantum memories, and quantum error correction or entanglement purification. These work in concert to reliably distribute entanglement (and thus quantum information) over extended distances. Below we explain each of these key technologies:
Entanglement Swapping
Entanglement swapping is the fundamental protocol that allows a quantum repeater to extend entanglement across multiple segments. It refers to the process of taking two independent entangled pairs and performing a joint measurement that “swaps” the entanglement, resulting in two previously unrelated particles becoming entangled. For example, imagine we have two entangled pairs: particles A–B entangled with each other, and particles C–D entangled with each other. B and C are at a middle repeater node (while A and D are at the distant end nodes). If the middle node performs a special joint measurement on particles B and C (a Bell state measurement), quantum mechanics will dictate that particles A and D become entangled – even though A and D never interacted directly​. In effect, the entanglement has been extended from the short A–B and C–D links into a longer A–D link, with the middle node acting as the swapping point. This is exactly how a repeater chains multiple segments: by entangling neighboring nodes, then “teleporting” the entanglement hop by hop until the end nodes share a state​. Entanglement swapping was first proposed in the 1990s as the linchpin of quantum repeater architectures, and it has since been demonstrated in labs as the way to connect two entangled links into one​. All prospective quantum repeater designs rely on entanglement swapping operations at intermediate nodes, typically implemented via Bell-state measurements (BSMs). The BSM consumes the two entangled pairs and projects the system into a new entangled state spanning the outer particles. Importantly, the outcome of the BSM can be communicated classically to the end nodes so they know their particles are entangled (this is called heralding). Thanks to entanglement swapping, quantum repeaters can create end-to-end entanglement without a direct physical connection between the end parties​. This concept has been vividly demonstrated with scenarios like “Alice, Bob and Charlie,” where Charlie (a middle node) performs a joint measurement that entangles Alice’s and Bob’s qubits even though Alice and Bob had no prior interaction​. Entanglement swapping is thus the basic “relay” mechanism of quantum repeaters, analogous to how classical repeaters relay bits – except here nothing is copied or amplified, only quantum correlations are extended.
Quantum Memories
One of the toughest technological requirements for a quantum repeater is having a reliable quantum memory at each repeater node. A quantum memory is a device that can store a quantum state (qubit) for a extended period without collapsing it. This is essential because entanglement distribution is typically a probabilistic and sometimes slow process – you may need to attempt sending entangled photons many times before success. With multiple segments, you need a way to hold on to the entanglement in one segment while waiting for the others to be established, so that entanglement swapping can be performed. Quantum memories serve this role​. When one segment (say between the first node and second node) successfully shares an entangled pair, those entangled qubits are stored in memory at the nodes. The repeater then waits until the next segment (second node to third node) also achieves entanglement. Once all adjacent links have entangled pairs ready in memory, the node in the middle performs the entanglement swapping measurement to connect the segments. Without memory, the first entangled pair would likely be lost (or decohere) while waiting for the second to succeed, especially as distances increase and success rates drop. Thus, quantum memories synchronize the entanglement distribution process, making it feasible to string together many short links into one long entangled connection​.
Implementing a good quantum memory is challenging. The memory must preserve the quantum state with high fidelity (minimal decoherence) for long enough to span the communication delays in the network. For instance, if two nodes are 100 km apart, even at light speed it takes about 0.5 milliseconds for signals to travel that distance; a repeater might need to hold a qubit for multiple milliseconds or more to coordinate multi-hop entanglement. In practice, researchers are pushing for memories with coherence times of seconds or longer. There have been notable achievements: e.g. trapped-ion memories have demonstrated coherence lifetimes over 30 minutes in the lab​, and atomic ensemble memories have achieved storage times on the order of milliseconds to seconds with reasonable fidelity. The main challenge is coupling these memories with photons – typically the qubits that travel are photons, which need to interact with the memory (usually an atomic system) to store the quantum state​. Efforts are ongoing with various platforms (trapped ions, neutral atoms in optical traps, doped crystal memories, NV centers in diamond, etc.) to create efficient light-matter interfaces. For instance, NIST scientists are developing ion-based quantum memories that can reliably catch and release photons at telecom wavelengths, aiming for memory spacing of ~30 km between repeaters​. Quantum memory is the cornerstone that distinguishes a true quantum repeater from simpler “entanglement swapping” experiments. With robust memories, a repeater node can perform successive entanglement swaps in a pipeline, effectively buffering quantum information like RAM in a classical network. Without memories, one can attempt so-called “all-photonic” repeaters (discussed below), but those require a huge overhead of photons and entangled resources. Thus, quantum memories are a critical technology to make quantum repeaters practical and scalable.
Quantum Error Correction and Entanglement Purification
Quantum information is notoriously fragile, so any realistic quantum repeater must deal with errors and noise that accumulate along the way. This is where techniques of quantum error correction (QEC) and entanglement purification come in. The basic entanglement swapping operation, when performed on real hardware, will yield an entangled state of the end nodes that is imperfect – loss and decoherence in the channels and at the nodes will degrade the fidelity of the entanglement. If the fidelity drops too low, the entangled state might not be useful for secure communication (for instance, QKD has a certain error rate threshold for security). To combat this, repeater schemes include methods to boost the quality of entanglement.
One approach is entanglement purification (also known as distillation). This is a protocol where two nodes share multiple low-fidelity entangled pairs and then apply judicious measurements and classical communication to “distill” a single pair with higher fidelity. Essentially, you sacrifice some of your noisy entangled links to filter out errors from one entangled pair. Purification can be done between neighboring repeater nodes to clean up entanglement before extending it further. Many first-generation quantum repeater proposals assumed entanglement purification would be used at each stage​. Purification, however, requires two-way classical communication between nodes and multiple entangled pairs, which can significantly slow down the effective rate of entanglement distribution. Still, it is a viable way to achieve high-fidelity end-to-end entanglement at the cost of throughput. In a repeater chain, one might generate a bank of entangled pairs on each segment, then run purification protocols to obtain a few ultra-high fidelity pairs, and only then use those for entanglement swapping to the next hop​.
Another approach (not mutually exclusive) is quantum error correction applied during the repeater process. Instead of using probabilistic purification with multiple copies, some repeater designs envision each node performing active quantum error correcting codes on the stored qubits. For example, a node could encode a qubit across several physical qubits in an error-resistant way, so that even if one photon is lost or one atom decoheres, the encoded logical qubit survives. There are proposals for one-way, all-photonic quantum repeaters that would send large entangled cluster states of photons which intrinsically correct losses on the fly using quantum error correction codes​. In those schemes, every repeater “node” might be just a station that performs measurements on a pre-entangled photonic state, effectively teleporting a protected logical qubit forward. The benefit is that no long-lived memory is required (photons carry the entanglement along), but the trade-off is an enormous overhead in number of photons and complexity of preparing error-correcting cluster states. Another trade-off is complexity: traditional repeater designs that use simpler memories and two-way communication are conceptually simpler but slower, whereas fully error-corrected one-way repeaters promise higher speed and longer reach but need more sophisticated quantum computing hardware at each node​.
In current experimental work, entanglement purification is the more immediately accessible technique – researchers have demonstrated the principle of distilling entanglement from a set of photon pairs. Quantum error correction at repeater nodes is likely a feature of “third-generation” quantum repeaters (a term used in some literature), which would require small quantum processors at each node to actively correct errors in real time. Whether via purification or QEC, the end goal is the same: ensure that the end-to-end entangled link has sufficiently low error (high fidelity) to be useful. Uncorrected, each link in a long chain will degrade entanglement quality, and after many hops the entanglement could become too noisy. The use of error correction techniques is therefore essential for scaling to truly long distances. Notably, a 2021 demonstration in a lab setting showed a quantum repeater node that achieved an improvement in key rate and error tolerance by effectively applying a form of entanglement swapping with two qubit memories – they managed to beat the error rate threshold (11% QBER) required for unconditional security by doubling the effective channel length, a proof that repeater-based error management works​. Ongoing research is refining these techniques so future repeaters can autonomously correct for photon loss, gate errors, and decoherence as quantum signals propagate. The combination of entanglement swapping, robust quantum memories, and error correction/purification protocols will ultimately allow quantum repeaters to deliver high-quality entanglement over arbitrarily long distances (in principle). These are the building blocks of tomorrow’s quantum internet infrastructure.
Comparison with Trusted Nodes
Because true quantum repeaters are still in development, many current long-distance quantum communication networks use a stopgap approach known as trusted nodes. It’s important to understand how the trusted-node scheme works and why it falls short of the ideal quantum repeater scenario in terms of security and scalability. Here, we compare the two approaches:
Trusted Nodes (Teleportation-by-Measurement) – In a trusted-node network (often called a “QKD relay network”), the route between Alice and Bob is divided into shorter spans connected by intermediate nodes. At each node, the quantum signal is measured, and a secret key is generated for that link (for example, using QKD over, say, 100 km). The node then stores the key and initiates a new QKD session on the next link, effectively acting as a translator between segments​. The final secret key between Alice and Bob is obtained by sequentially combining (e.g. XOR-ing) the keys from each segment. The critical point is that each intermediate node knows the keys for its two adjacent links, so it can recombine them. Thus, you have to trust each node not to be compromised, since a malicious node could read the key or leak it. China’s 2,000 km QKD network, for example, employed over 30 trusted relay nodes, each housed in a secure location, to link Beijing and Shanghai​. This allowed banks and government sites along the route to exchange quantum-generated keys, but if any one of those nodes were breached, the security of keys passing through it would be lost​. In practice, these nodes are in controlled facilities with authentication, so the system’s security reduces to classical security at those points. Trusted nodes effectively break a long quantum path into many independent QKD links.
Quantum Repeaters (Entanglement-Based) – A network based on true quantum repeaters would look very different. Instead of measuring and generating new keys at each node, a quantum repeater node never has to learn any key or quantum data. It merely facilitates entanglement between the neighboring links (via entanglement swapping as described). The end result is that Alice and Bob share one continuous quantum link and can perform QKD end-to-end. The intermediate nodes do not possess the final key at any point – in fact, they don’t even possess a copy of the qubits’ state. They only perform quantum operations (like Bell measurements) that refresh the entanglement and forward it along​. This means even if an adversary controlled a repeater node, they could not steal the key, because the key is generated only when Alice and Bob measure their entangled qubits at the very end. A true quantum repeater network thus restores the unconditional security promise of QKD across long distances. As one expert noted, “One way to reduce the risk of relying on trusted nodes is to introduce quantum repeaters at each relay point. Such repeaters…allow the signal to be refreshed and passed on directly without having to store the key bits temporarily.”​ In other words, quantum repeaters eliminate the need to trust intermediate nodes – security is guaranteed by quantum physics from end to end, just as it is in a simple point-to-point QKD link, but now over global distances.
Beyond security, scalability and network complexity are affected by these approaches:
- Security: Trusted nodes create multiple points of vulnerability (each node must be physically secure and trusted personnel must operate it). Quantum repeaters remove these vulnerabilities by design – there is no secret information at the nodes to protect​. This makes a repeater-based quantum network conceptually closer to the ideal of unhackable communication. However, repeater nodes will still need tamper-proof hardware to ensure an attacker doesn’t alter their operation (e.g. forcing them to perform measurements). But even if a repeater node were hacked, the worst it could do is disrupt the service (a denial of service); it could not undetectably siphon off the key. By contrast, a hacked trusted node can silently copy the key without Alice or Bob knowing.
- Reliability: In a long chain of trusted nodes, the failure of one node breaks the chain, and the network must rely on classical fail-safes. Quantum repeater networks can similarly be disrupted if a node fails, but interestingly, future quantum networks might allow multipath entanglement routing – analogous to Internet packet routing – where if one repeater path is down, another path of entangled links could be used. This is only feasible when many quantum repeaters are interlinked in a mesh, a capability that trusted-node schemes (which require fixed point-to-point trusted relationships) might struggle with.
- Scalability: Trusted node networks have been demonstrated at nationwide scale (China’s network spans 4,600 km when including satellite links)​. But scaling further (or connecting networks internationally) means exponentially more nodes and complex key management between every pair of nodes. Each node adds latency because keys must be generated link by link. In contrast, if one could deploy quantum repeaters every ~50–100 km, the same QKD protocol could, in principle, extend indefinitely by chaining entanglement, with only linear increase in time due to communication and possibly some logarithmic increase due to purification protocols. Quantum repeaters also operate at the quantum level, so once set up, the key can be established with fewer rounds of communication compared to managing many intermediate keys.
- Use Cases: Trusted nodes suffice only for key exchange (and then only if you trust them). They cannot distribute entanglement itself beyond one link. That means you cannot do things like entangle two distant quantum sensors via a trusted node – the node would measure (destroy) the entanglement. Quantum repeaters enable new functionalities: remote entanglement-based clock synchronization, distributed quantum computing (where two remote qubits interact via entanglement), quantum voting protocols, etc. These are beyond the scope of trusted-node networks. In terms of future-proofing security, trusted nodes are something of a stopgap; they forego the unconditional security of pure quantum links to achieve distance​. Quantum repeaters aim to deliver both distance and unconditional security.
In summary, trusted nodes vs. quantum repeaters is analogous to the difference between sending a secret message by courier through many fortified castles (hoping none of the couriers or lords betray you) versus sending it via a sealed unbreakable pipeline that runs directly to the recipient. The “pipeline” of course is harder to engineer (we’re in the midst of that engineering challenge now), but once built, it offers far greater security. Trusted nodes have been invaluable for early quantum network trials, but they are inherently limited. The long-term vision – often called the Quantum Internet – relies on true quantum repeaters so that information can be transmitted with quantum-secured end-to-end encryption across arbitrary distances​. Achieving this will remove the need for intermediate trust and allow quantum networks to scale much like classical networks, but with superior security guarantees.
Current Status of Quantum Repeater Development
Quantum repeater technology is rapidly progressing in research labs, though not yet at the level of widespread practical deployment. As of 2024, we are seeing a series of proof-of-concept demonstrations and incremental milestones that indicate steady progress toward functional repeater-based networks. Both academic institutions and private companies are deeply involved in this effort, and governments have launched major initiatives to support the development of a global quantum network.
On the academic front, one of the landmark achievements came in 2021 from the Netherlands: QuTech at Delft University demonstrated the first multi-node quantum network, entangling three remote quantum processors (nicknamed Alice, Bob, and Charlie) in different labs​. In that experiment, they used nitrogen-vacancy (NV) centers in diamond as the quantum nodes and even incorporated a memory qubit at the middle node (Bob) to perform entanglement swapping between Alice and Charlie. This was effectively a rudimentary quantum repeater scenario – entanglement was created between Alice–Bob and Bob–Charlie, and then swapped so that Alice and Charlie became entangled​. Experts hailed this as “an important milestone in the quest for the quantum Internet”, demonstrating entanglement swapping in a real network of more than two nodes​. It was still on a lab scale (the nodes were 1.3 km apart within Delft), but it showed that a small quantum network with a repeater-like node is feasible.
Around the same time, significant progress was made in China. In 2020, a team led by Prof. Jian-Wei Pan at USTC (University of Science and Technology of China) achieved entanglement between two quantum memories over a 50 km fiber link, smashing the previous record of 1.3 km for memory-to-memory entanglement​. They entangled two atomic ensembles (quantum memories) via fibers and were able to maintain entanglement at “city scale” distances. Pan noted that “the main significance of this paper lies in extending the entangling distance between quantum memories to the city scale”​. This was a major step toward practical repeaters because it combined photons and memory-based qubits in a long fiber experiment. While this result still relied on laboratory conditions (coiled fiber in a lab), it demonstrated the ability to distribute entanglement across metropolitan distances with quantum memory – a prerequisite for repeaters. China has been a leader in quantum communication; they also launched the Micius satellite in 2016 and integrated satellite QKD with their 2,000 km fiber network​. However, that fiber network used trusted nodes. The 50 km memory entanglement experiment is more directly a repeater breakthrough, pointing toward eventually replacing those trusted nodes with true quantum repeater links.
In Europe, another cutting-edge experiment occurred in 2023: researchers at the University of Innsbruck (Austria) and Paris-Saclay (France) unveiled a prototype quantum repeater system that combined all key functionalities in one setup and successfully transmitted entanglement over 50 km using an intermediate repeater node​. In their experiment, they used two trapped-ion nodes as the endpoints and a fiber link of 50 km between them (actually two 25 km spools). Each ion emitted an entangled photon; these photons were frequency-converted to telecom wavelength (1550 nm) for low-loss transmission and sent to two remote fiber nodes​. At those nodes, the photons’ arrival triggered the ions’ states to be stored in long-lived memory states​. By repeating this process and performing a Bell-state measurement, they effectively teleported quantum states between the ions across 50 km. Notably, this demonstration ticked several important boxes: telecom-compatible photons, quantum memory storage, and entanglement swapping with heralding, all achieved together​. The success rate was still low (they reported about 2,053 successful entanglement events out of ~2.2 million attempts over 33 minutes) but the use of memory made entanglement 128 times more likely than it would be without memory buffering​. The team is now planning to move out of the lab and test their system in real deployed fibers between cities – “Our vision is to get out of the lab and start building quantum networks of matter and light between cities and countries,” said one researcher​. This experiment is arguably the clearest glimpse yet of a working quantum repeater node under real-world conditions.
The United States has also made notable strides. In 2020, scientists from Argonne National Lab and University of Chicago entangled photons across a 52-mile (84 km) fiber loop in the Chicago area, one of the longest land-based quantum link tests at the time. This was part of the U.S. Department of Energy’s quantum internet program, and it established a testbed for entanglement distribution in real-world conditions (the fiber ran under suburban streets). While that specific demonstration was an entangled-photon link (Bell pair) over 84 km rather than a multi-hop repeater, it set the stage for testing quantum communication hardware on installed fiber infrastructure​. In late 2020, Fermilab and partners announced a milestone in quantum teleportation: they achieved sustained quantum teleportation of qubits over a 44 km fiber network with >90% fidelity​. This relied on entanglement distributed across two laboratories and is another piece of the repeater puzzle (teleportation is essentially what a repeater does, teleporting qubits across segments). Building on these developments, the U.S. has laid out a “quantum internet blueprint” and established test networks connecting national labs and universities (e.g., the Illinois Express Quantum Network linking Argonne, Fermilab, and Northwestern). These networks are initially using entangled photons and some trusted nodes as needed, but they are poised to incorporate new repeater technologies as they mature.
Several startups and private companies are also driving quantum repeater development. A notable example is Qunnect, a U.S.-based startup focusing on commercial quantum networking equipment. In 2023, Qunnect and researchers at New York University successfully tested a 10-mile (16 km) quantum link across New York City – from the Brooklyn Navy Yard to Manhattan – using entangled photonic qubits distributed at 15,000 pairs per second with 99% uptime over existing telecom fiber. This was done with Qunnect’s “GothamQ” testbed and is essentially a metropolitan quantum network demo. While this particular test did not yet deploy a multi-hop repeater (it was an entangled photon source delivering pairs to two distant sites), Qunnect is developing quantum memory and entanglement swapping hardware as part of its product suite. In fact, Qunnect has one of the first commercial quantum memory devices (the “Qu-Memory”), and it envisions an integrated quantum repeater product that will use memory and entanglement swapping to extend entanglement beyond the direct fiber length​. The successful 16 km urban test shows that quantum entanglement distribution can be made robust in a noisy city environment – a promising sign for real-world deployment​. Companies like Qunnect, along with others (e.g., QuintessenceLabs, ID Quantique, Toshiba) are working on pieces of the quantum network puzzle, from quantum memory and sources to the classical networking needed to control these systems.
Large tech players are involved too. Amazon AWS established the AWS Center for Quantum Networking (CQN), and in 2023 AWS partnered with Harvard University to demonstrate a quantum network over 35 km of deployed fiber in the Boston area​. This experiment used silicon-vacancy centers in diamond as quantum memories and achieved entanglement between two memory nodes with a photon that traveled out-and-back through metropolitan fiber and was stored for over a second​. The ability to maintain entanglement for >1 second over tens of kilometers is a big technical win, as it shows long-lived solid-state memories can interface with real-world fiber links. AWS’s team described this as the first demonstration of entanglement of two quantum nodes across a deployed fiber network, signaling that quantum repeaters (or at least the memory + entanglement distribution aspect) are leaving the lab environment​. Similarly, Cisco has a quantum networking research group (as evidenced by their 2025 blog posts on quantum repeaters) working on repeater protocols and hardware integration​. Google, IBM, and Microsoft are more focused on quantum computing, but IBM has done some quantum networking research and companies like Honeywell/Quantinuum (with trapped-ion technology) could leverage their systems for networking experiments as well.
In the public sector, NIST (USA) is actively researching quantum repeaters, focusing on trapped-ion memories and neutral-atom memories. They have demonstrated impressive memory times and are developing transducers to convert trapped-ion qubit states to telecom photons​. The European Union’s Quantum Flagship program has a Quantum Internet Alliance that includes groups from Delft, Paris, Geneva, and others, working toward a blueprint for a pan-European quantum network. There are testbeds in the EU connecting cities (for example, a recent test connected Delft, The Hague, and Amsterdam with an entangled link of 25 km using existing fiber​). Japan and Canada also have quantum communication test networks. As of now, no fully operational quantum repeater network exists – i.e., there is no network where entanglement is stored and swapped over multiple hops in a routine, commercial manner. But experimental demonstrations have achieved key repeater milestones: entanglement swapping between independent links​, memory-enhanced entanglement distribution over tens of kilometers​, metropolitan field tests of entangled photons​, and even prototype repeater nodes performing better than direct transmission (as shown by a 2021 experiment that beat the direct-loss key rate using a single repeater node with two atoms)​.
In short, the current status is that quantum repeaters are transitioning from theory to practice. We have isolated demonstrations of all the necessary pieces: long-lived quantum memories, entanglement swapping operations, entanglement over long distances, and even small quantum networks. The challenge now is engineering these components into integrated systems that can operate outside a physics lab. Organizations around the world are heavily invested in this – from university labs pushing the science, to startups building hardware, to government-funded networks acting as testbeds. A 2021 position paper from German BSI summed it up: “Quantum repeaters are still the subject of fundamental research and not practical at present.”​ That is true today, but the gap between research and practical use is closing year by year. If the current pace of progress continues, we can expect pilot quantum repeater networks to emerge in the coming years, first on city-to-city scales, then scaling up as technology matures.
Cybersecurity Implications of Quantum Repeaters
For cybersecurity professionals, quantum repeaters and the quantum networks they enable carry both promising security benefits and new considerations in terms of threat models and infrastructure. On the whole, quantum repeaters stand to greatly enhance security in communications by extending the reach of quantum cryptography. But like any technology, they also introduce new components that must be secured and potential attack vectors that need to be understood. Here, we examine the implications:
Enhanced Security Through Quantum Networking
The primary cybersecurity benefit of quantum repeaters is that they facilitate cryptographic key exchange with unconditional security over long distances. In a post-quantum world – where quantum computers could break classical public-key algorithms – QKD offers an alternative based on the laws of physics rather than computational assumptions. With repeaters, QKD is no longer limited to short distances. This means organizations could, for example, share one-time-pad keys between distant data centers or offices in different cities, with the guarantee that no eavesdropper (no matter how powerful) can intercept those keys without being detected. Quantum cryptography’s security is founded on principles like the uncertainty principle – any interception disturbs the quantum state and can be noticed by the communicating parties​. By enabling QKD on a global scale, quantum repeaters allow us to replace vulnerable classical key exchanges (which could be recorded and later decrypted by a quantum adversary) with information-theoretically secure exchanges. This is a profound shift: even an attacker with unlimited computing power cannot break the keys delivered by an ideal QKD network with repeaters​. In contrast, post-quantum cryptographic (PQC) algorithms (the classical alternative to QKD) are based on mathematical problems that we believe to be hard, but this security is conjectural. QKD, supplemented by repeaters, provides a level of security assurance that is fundamentally different – it’s provably secure under the laws of quantum mechanics​. In practice, it means communications could be secured against future threats (including quantum computers and unforeseen advances in cryptanalysis) by relying on keys generated via quantum networks. This addresses the “store-now, decrypt-later” risk in current encryption: even if an adversary records your encrypted traffic today, they won’t ever be able to decrypt it if the keys came from a quantum-secure method​.
Another implication is that quantum networks can enable new security protocols that have no classical analog. For example, quantum entanglement can be used for device-independent quantum key distribution, where even if your devices are untrusted or built by an adversary, you can still ensure security by testing quantum correlations (a very advanced concept, but potentially important for high assurance applications). Such protocols typically require entanglement shared over long distances – something repeaters would provide. Quantum repeaters also make it feasible to implement protocols like quantum secret sharing (splitting a secret among multiple parties such that only certain groups can reconstruct it) and quantum secure direct communication (sending data directly over an entangled link without a key, with inherent eavesdropping detection). These protocols offer specialized security advantages (e.g., protection even if some nodes are compromised) and are areas of active research.
In summary, quantum repeaters extend the quantum security advantage to the scale of real networks. They allow the creation of a quantum-secure backbone that could connect data centers, financial institutions, government facilities, etc., ensuring that even nation-state adversaries cannot eavesdrop on critical communications. This doesn’t eliminate the need for classical cybersecurity (one still needs authentication, access control, etc.), but it provides a powerful additional layer. In practice, we will likely see hybrid cryptographic infrastructures: for instance, using quantum-generated keys to frequently refresh symmetric encryption keys in classical networks, alongside post-quantum algorithms as a backup​. For a CISO, the advent of quantum repeater networks means there’s a potential new tool to achieve security goals that were previously out of reach – like absolutely secure long-haul links for sensitive data, or an ability to detect any interception on a network line. It’s a way to future-proof communications against not just quantum computing threats but any computational attack, since the security is not based on math problems but on physics that has been validated for decades.
Potential Vulnerabilities and Attack Vectors
While the theoretical security of repeater-based QKD is ironclad, the practical security depends on the hardware and implementation. Cyber adversaries will undoubtedly look for ways to exploit the real-world imperfections in quantum repeater systems. Indeed, history shows this is likely: researchers have already found loopholes in QKD systems (without repeaters) – for example, the notorious detector blinding attack where an eavesdropper shines a bright light to control the single-photon detectors, converting the quantum channel into a classical-like channel without being detected​. This “perfect eavesdropper” attack demonstrated that if an attacker can tamper with the physical devices (or their settings), they might break the security of quantum protocols without triggering the usual alarms, by exploiting technical weaknesses rather than breaking quantum physics. Manufacturers like ID Quantique had to patch their QKD systems to close such loopholes​.
In a quantum repeater network, we now have more components that could be targeted: photon sources, quantum memories, the Bell-state measurement devices, and the classical synchronization channels between nodes. Some potential attack vectors and challenges include:
- Compromised Repeater Node: If an attacker gains access to a repeater node, could they undetectably siphon information? In principle, if the repeater is functioning correctly, it never has the full key or message. However, a malicious actor could modify the repeater’s behavior – for instance, secretly perform measurements on qubits passing through (turning it into a “trusted node” without trust). This would introduce detectable disturbances if done crudely, but a sophisticated adversary might try to perform something like an intercept-resend at the quantum level, or manipulate the error rates just below the alarm threshold. Ensuring physical security and integrity of repeater stations is thus crucial. This is similar to protecting classical network infrastructure from tampering, but with the twist that even analog optical components and precise timing systems must be secured against interference.
- Side-Channel Attacks: A quantum repeater, especially one using quantum memories, might inadvertently leak information through side channels. For example, a quantum memory might emit or absorb photons that an eavesdropper could monitor (if the memory is, say, an ensemble of atoms, their state could slightly affect some optical properties). A repeater’s physical hardware might also betray timing information – e.g., an outside party might detect when a Bell-state measurement has succeeded by sensing a faint vibration or a power surge. Such side channels are speculative, but adversaries are very creative at finding unintended signals. Security testing for quantum network devices will need to be as rigorous as pen-testing classical systems, extended to the quantum domain.
- Denial-of-Service (DoS): It’s relatively easy to foil a quantum communication link by introducing noise. An attacker could simply inject bright light into the fiber to drown out single-photon signals, or use a laser to knock qubits out of their quantum states. Quantum repeaters won’t necessarily help if someone is jamming the quantum channel – they too rely on low-noise quantum signals. Thus, quantum networks will need classical failovers or at least detection mechanisms for jamming. An attacker might not be able to read the traffic, but they can disrupt it. From a cybersecurity standpoint, ensuring availability (one of the CIA triad: confidentiality, integrity, availability) remains as important as confidentiality. Redundant paths and perhaps classical fallback modes will be needed to mitigate DoS on quantum links.
- Classical Channel Attacks: Quantum repeaters also use classical communication (for example, to acknowledge entanglement success or to initiate purification protocols). These classical channels could be hacked in typical ways – e.g., spoofing a signal to make one node believe entanglement succeeded when it didn’t, potentially leading to synchronization errors or even security weaknesses if not handled properly. All classical communications in a quantum network must be authenticated (often with pre-shared symmetric keys or PQC algorithms) to prevent man-in-the-middle manipulation​. If, say, an attacker injected fake signals that say “I successfully shared entanglement with you” to two distant nodes, they might trick them into thinking they share a key when they don’t, causing confusion or false sense of security. Rigorous protocol design and authentication is therefore required.
- Software and Control: The control systems orchestrating a quantum repeater network (the software that tells lasers when to fire, that performs error correction algorithms, etc.) are classical computers which could be targeted by malware. The quantum part might be secure, but if an attacker infiltrates the network control software, they might cause it to misbehave (e.g., using poor random numbers, or intentionally leaking key bits via the public discussion channel). Standard cybersecurity best practices will need to apply: secure software development, regular audits, defense-in-depth.
The good news is that the quantum cryptography community is aware of these issues and is actively developing countermeasures. For instance, improved detector designs that are immune to blinding attacks have been developed​, and researchers classify possible quantum network attacks to design defenses​. Quantum repeaters themselves can also incorporate verification steps: e.g., the end users can perform random checks on entangled pairs (sacrificing a small subset to test for Bell inequality violations) to ensure no one has tampered with the entanglement distribution. If the observed quantum correlations fall below what’s expected, that could indicate an attack or faulty node. This is analogous to the test in QKD where a portion of bits are compared publicly to estimate an error/eavesdropping rate​.
Finally, we should consider integration with post-quantum cryptography. It’s likely that for the foreseeable future, organizations will adopt a hybrid approach: using both PQC algorithms (like lattice-based encryption) and QKD (where available) to secure communications. Quantum repeaters, by extending QKD, make this hybrid approach viable over long haul links. The implication is that network architects will have to manage two parallel infrastructures: one classical (but upgraded to PQC algorithms) and one quantum. Each has different failure modes – PQC could be broken if, hypothetically, someone finds a mathematical weakness, whereas QKD could fail if hardware is compromised. Using them together could provide defense in depth. For example, one might encrypt data with a combination of a one-time pad (key from QKD) and a PQC cipher – an attacker would need to break both the physics and the math, an extremely tall order. On the flip side, the deployment of quantum networks will require new standards, key management systems, and interoperability frameworks, which cybersecurity professionals will need to develop and scrutinize. Ensuring the post-quantum cryptographic infrastructure and the quantum network infrastructure complement each other is a challenge that will define the next era of secure communications. The bottom line: quantum repeaters promise unparalleled security for data in motion, but they come with new hardware that must be secured against novel attacks. With careful design, monitoring, and blending with classical security measures, the risks can be managed, allowing us to reap the security benefits.
Future Prospects and Challenges
The path toward a fully-fledged quantum repeater network – a quantum internet – is exciting but full of challenges. Looking ahead, we can outline the expected advancements that researchers and engineers are striving for, as well as the hurdles they must overcome to deploy quantum repeaters on a global scale.
Advancements on the Horizon:
- Higher-Performance Quantum Memories: We expect continuous improvement in quantum memory duration, efficiency, and bandwidth. Today’s best memories (like certain ion traps or cryogenic solid-state systems) can store qubits for minutes with high fidelity​, but practical repeaters might need memories that are also fast – able to write and read qubits quickly to keep up with communication rates. Efforts like integrated photonic memory modules, broadband atomic memories, and microwave-to-optical transducers for superconducting qubits all aim to make quantum memory more versatile. A key milestone to watch for is a memory that can simultaneously achieve long storage time, work at telecom wavelength, and have high write-read efficiency (>90%). Such a memory would be a game changer for repeater designs.
- Better Entanglement Sources: Similarly, entangled photon sources will improve. Future repeaters will benefit from sources that can generate entangled pairs at higher rates (millions of pairs per second or more) with multiplexing – the ability to try many entanglement attempts in parallel. This could involve frequency multiplexing (many frequencies of light in the same fiber) or spatial multiplexing (many cores in one fiber), combined with memories to sort out which attempt succeeded. High-rate entanglement distribution will raise the end-to-end secure key rates of quantum networks, making them more practical for large-volume data protection.
- Second- and Third-Generation Repeater Protocols: The first generation of quantum repeaters (as originally proposed by Briegel et al.) relied on heralded entanglement and two-way communication for purification. Second-generation protocols aim to use forward error correction to reduce the need for two-way handshakes, and third-generation would use full quantum error correction and one-way transmission of encoded states. In the coming years, we expect theoretical and experimental progress on all-photonic repeaters (which encode qubits in large photonic cluster states to circumvent memories)​ and error-corrected repeater nodes (where each node is a small quantum computer correcting errors on the fly). A recent Cisco research post, for instance, discussed a design for a one-way photonic repeater that emphasizes simple error-correcting operations at each node​. While these advanced approaches are still in the lab, they hint that future repeaters might not even need to wait for classical signals between nodes, which would greatly speed up quantum communication. By around 2030, we may see prototypes of such one-way repeaters demonstrated in test networks.
- Scaling Up Demonstrations: In the near future, expect to see city-to-city quantum repeater testbeds. For example, the EU Quantum Internet Alliance aims to connect four Dutch cities in a quantum network testbed in the mid-2020s. Similarly, in the U.S., groups could connect campuses or cities (e.g., Boston to New York quantum link, or Los Angeles quantum network). These testbeds will likely involve a handful of repeaters (maybe one or two hops to start) and will serve as blueprints for larger networks. Each success will bring valuable learning about real-world issues like fiber noise, environmental fluctuations, and integration with existing telecom infrastructure.
- Standardization and Interoperability: Another prospect is the development of standards for quantum networking. Organizations like the ITU and IEEE have begun discussing quantum network standards (for QKD interfaces, etc.). As repeaters become reality, standard protocols for entanglement swapping, error rates, handshaking, and even quantum network routing will emerge. Industry consortia may agree on how to represent a qubit over fiber (e.g., using specific wavelengths or encoding schemes) so that different vendors’ equipment can interoperate. This will mirror the standardization process that happened for the classical Internet, albeit in a very specialized domain.
Challenges Ahead:
Despite the progress, significant challenges remain before we have a global quantum repeater network:
- Loss and Distance: Optical fiber has a fixed attenuation (~0.2 dB/km at 1550 nm). Quantum repeaters can leapfrog over loss, but each repeater node introduces its own loss and error probabilities. Placing repeater nodes at optimal intervals (perhaps every 50–100 km for fiber) will be necessary. That means for a transcontinental link of 1000 km, you might need 10–20 repeater nodes in between. Each node is a complex quantum device, far more complicated (and expensive) than a classical amplifier. Rolling out this infrastructure is a massive engineering effort. Additionally, connecting distant continents likely requires satellites or undersea fiber. Satellites can provide entanglement between ground stations thousands of kilometers apart (China has shown satellite-mediated entanglement over 1200 km​), potentially reducing the number of ground repeaters needed. But integrating satellite links (which are intermittent and affected by atmosphere) with fiber repeaters is nontrivial.
- Reliability and Error Rates: Current quantum hardware is not as reliable as classical gear. A memory might fail to capture a photon 30% of the time, or a gate operation might have a 2% error. In a chain of many repeaters, these imperfections multiply. To scale up, quantum repeaters must become orders of magnitude more reliable in their operations, or have robust error correction to compensate. This is essentially the same challenge faced in building quantum computers: error rates must be lowered or corrected for. Research by Pan’s group noted that improvements in entanglement fidelity, gate operations, and storage are needed before large-scale networks can be constructed​. This is a physics and engineering problem: better materials, better isolation from noise, and perhaps newer quantum systems (like error-corrected superconducting qubits or photonic qubits) might be required.
- Manufacturing and Cost: Right now, each quantum repeater demonstration is a physics experiment with custom-built components. To deploy hundreds or thousands of repeaters, we will need commercially manufacturable quantum hardware. This includes sources (entangled photon-pair generators, single-photon sources), detectors (high-efficiency, low-noise single-photon detectors that can operate 24/7), stable lasers, quantum memory modules, etc. The cost of these must come down with economies of scale. It’s telling that some startups are already packaging parts of this – for instance, Qunnect sells a room-temperature quantum memory device, and other companies offer turn-key QKD systems. As technology matures, we’ll likely see rack-mount quantum repeater units that telecom companies or governments can deploy at repeater stations. But reaching that point requires a lot of engineering refinement and investment.
- Integration with Classical Infrastructure: A practical challenge is how to integrate quantum channels with existing fiber networks. Most likely, quantum signals will co-propagate in the same fiber bundles as classical signals (to avoid laying completely new fibers). This can introduce cross-talk (classical signals might create noise for the quantum signals). Techniques like wavelength multiplexing and filtering are needed to allow quantum and classical communications to share infrastructure without interference. Furthermore, placing quantum equipment in telecom huts or satellites means dealing with power, maintenance, and environmental conditions (temperature fluctuations can disturb delicate quantum optics). Ensuring that quantum repeaters can operate robustly in the field – possibly unmanned – is a big hurdle. They might need advanced auto-calibration and remote management systems.
- Timeline for Deployment: When will we see a real quantum repeater network? Optimists point to the rapid progress and suggest we could have regional quantum networks with repeaters by the later 2020s. Indeed, Europe’s quantum internet roadmap aims for a prototype with multiple quantum repeaters within this decade. Pessimists (or realists) note that it may take longer for the technology to be as dependable as needed – perhaps the 2030s for large-scale deployment. It’s instructive to recall that the basic concepts were proposed in the late 1990s, and only now are we seeing multi-node networks in the lab. Scaling from those experiments to a full network is an engineering project that could easily span 10–20 years. We might see a global quantum network by 2040 if progress continues, potentially earlier if there are breakthrough innovations or strong economic push. On the other hand, simpler QKD networks using trusted nodes and satellites will fill the gap in the meantime, as they already do in certain areas.
- Skilled Workforce and Interdisciplinary Know-How: Building and maintaining quantum networks will require quantum physicists, engineers, and cybersecurity experts working together. There is a challenge in growing the workforce with the right skill sets. People need to understand both quantum mechanics and networking. This is as much a human challenge as a technical one – training programs (like new university courses on quantum networking) are starting to emerge to address this.
In summary, the future of quantum repeaters is bright but demanding. We anticipate steady breakthroughs: longer distances achieved with fewer errors, more nodes entangled in bigger networks, and eventually early versions of a quantum internet spanning multiple cities or countries. With each milestone – a 1000 km entanglement, a 10-node network, a day-long stable operation – the vision comes closer. The challenges of scaling, standardizing, and securing the technology are significant, but not insurmountable. Importantly, as the threat of quantum computers to classical cryptography grows, there is increasing motivation (and funding) to solve these challenges. Governments are investing hundreds of millions into quantum communication R&D, recognizing that a quantum-secure communication infrastructure could become as critical as classical cybersecurity is today.
The timeline may unfold in stages: first, local quantum networks (within cities or between nearby research sites) demonstrating repeater functionality by 2025; next, metro-scale and inter-city networks (hundreds of km, with a few repeater hops) by around 2030, possibly serving early adopter industries (banking, defense) on a limited scale. Beyond that, in the 2030s, national and international quantum networks could take shape, combining fiber repeaters, undersea quantum links, and satellites to cover the globe. Each stage will likely coexist with classical networks, gradually integrating. It’s worth noting that intermediate technologies like satellite QKD might connect continents before fiber repeaters do – for instance, satellite links could cover oceans while repeater chains cover land. By the time all these are connected, we will have the backbone of a global quantum internet.
Conclusion
Quantum repeaters are the linchpin technology for the next revolution in secure communications. They address the fundamental limitations of direct quantum communication by introducing a way to extend entanglement over arbitrary distances, enabling protocols like QKD to work from neighborhood scales to intercontinental scales with the same strong security grounded in quantum physics. In this article, we’ve explored what quantum repeaters are – essentially, quantum devices that perform the equivalent of signal boosting through entanglement swapping and quantum memory – and why they are so important. We’ve seen that without repeaters, we’re forced to trust intermediate nodes or accept limited range, both of which undermine the promise of quantum cryptography. Quantum repeaters, by contrast, restore end-to-end quantum security, making them critical for future quantum networks and a quantum internet.
We delved into the key technologies that make repeaters possible: entanglement swapping (teleporting quantum states across network hops), quantum memories (storing qubits to synchronize entanglement distribution), and error correction techniques (to overcome noise and loss). These are active areas of research, each progressing steadily. We compared the current workaround – trusted node networks – with the ideal repeater-based networks, highlighting how repeaters eliminate the need to trust intermediate points and offer better long-term scalability and functionality. We reviewed the current state of development: from lab experiments entangling memories tens of kilometers apart​, to multi-node network demos​, to field tests in city fibers​. The progress in the last few years alone has been remarkable, indicating that the pieces of a real quantum repeater network are coming together. Initiatives across the globe, involving universities, government labs, startups, and big tech companies, are all pushing this technology forward.
For cybersecurity professionals, quantum repeaters carry big implications. On one hand, they herald a future where truly secure key exchange is possible over the entire globe – a powerful tool against surveillance and cyberattacks, even those backed by quantum computers. On the other hand, they introduce new hardware and complex quantum protocols that must be integrated into the security landscape. We discussed how repeaters enhance security and also what new attack vectors they might present (like hardware side-channels or denial-of-service risks), emphasizing that careful engineering and a defense-in-depth mindset remain crucial. Quantum networks will augment, not entirely replace, classical security; they will work alongside post-quantum cryptography to protect data. The advent of quantum repeaters essentially adds a “quantum tier” to cybersecurity – one that, if implemented right, can significantly raise the bar for attackers.
Looking to the future, we expect quantum repeaters to improve rapidly. Many challenges lie ahead in scaling the technology and making it robust, but none appear insurmountable. The timeline for a functional quantum repeater network (beyond experimental testbeds) might be on the order of a decade or more, but each intermediate achievement will likely find niche applications (for example, ultra-secure links for government communications). The vision of a global quantum internet – where quantum information can be sent securely and even quantum processors in different cities can be entangled – is driving these efforts. Achieving that vision will likely require continued investment, not just in research but in infrastructure deployment. Governments and industry stakeholders will need to commit resources to build pilot networks, much like ARPANET was a pilot for the internet.
In conclusion, the development of quantum repeaters represents a convergence of quantum physics and network engineering that could redefine how we secure information. The key takeaways are: quantum repeaters enable long-distance quantum communication by solving photon loss and no-cloning challenges​; they are essential for expanding QKD and other quantum protocols globally, far beyond the limits of direct transmission​; they rely on advanced technologies like entanglement swapping, quantum memories, and error correction to function​; they offer clear advantages over current “trusted node” methods in terms of security (no more intermediate key exposure) and eventually cost-efficiency and flexibility​; and while still in the experimental stage, rapid progress is being made by leading labs and companies to bring them to reality​. For cybersecurity, this means preparing for a paradigm shift where quantum keys and entangled qubits become part of the network toolkit, and ensuring the new quantum infrastructure is itself secure.
The importance of continued investment and research in quantum repeaters cannot be overstated. As quantum computers advance, so does the urgency of deploying quantum-safe communication methods. Quantum repeaters are the most promising route to achieve quantum-safe communications at scale. Each dollar and effort spent now on overcoming the scientific and engineering challenges will pay off in the form of communications networks that can stand up to any computational threat. Moreover, leadership in quantum communications may translate to geopolitical and economic advantages, as secure information exchange is a cornerstone of national security and finance. We are witnessing the early days of what might eventually become a parallel network layer – a quantum layer – atop the classical internet. Just as the development of classical repeaters and fiber optics revolutionized global connectivity in the late 20th century, the maturation of quantum repeaters could revolutionize security and capabilities of networks in the 21st. In a future not too far off, it’s conceivable that when two distant offices establish a secure connection, under the hood quantum entanglement via a chain of repeater nodes will be setting up the encryption keys – instant, unbreakable, and oblivious to any eavesdropper. That is the promise driving quantum repeater research. And with sustained effort, that promise will be fulfilled, ushering in the era of the quantum-secure internet.