Quantum Computing Modalities: Spin Qubits in Other Semiconductors & Defects
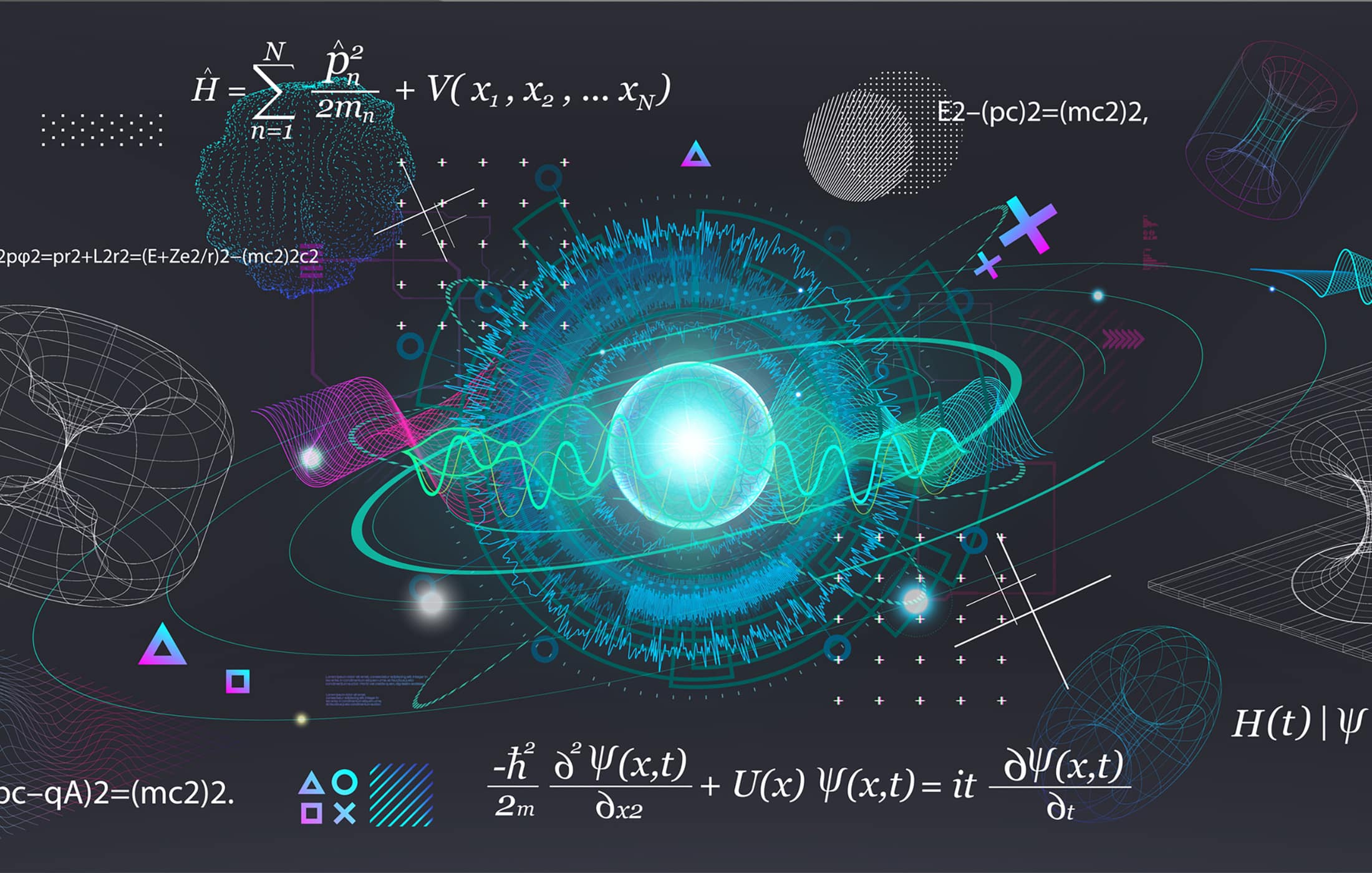
Table of Contents
(For other quantum computing modalities and architectures, see Taxonomy of Quantum Computing: Modalities & Architectures)
(Note: There is some overlap with silicon-based qubits, but here we include other spin-qubit implementations: in III-V semiconductor quantum dots, in diamond NV centers, etc., highlighting approaches outside silicon or in “exotic” materials.)
What It Is
In addition to silicon, spin qubits can be realized in other solid-state systems. One well-known example is the nitrogen-vacancy (NV) center in diamond, which is a point defect where a nitrogen atom next to a vacancy in the carbon lattice creates an electronic spin-1 system that can be used as qubit (often using the $$m_s=0$$ and $$m_s=+1$$ sublevels as |0⟩ and |1⟩). NV centers have the unique ability to be controlled and read out even at room temperature by optical means (they fluoresce bright or dim depending on spin state under green laser excitation). They also have a nuclear spin (like the N’s nuclear spin) that can serve as auxiliary qubits. NV centers and similar defects (like silicon vacancy in diamond, divacancies and single silicon carbide defects, etc.) are pursued for quantum networking (as single-photon sources) and for quantum computing nodes (e.g., small registers of a few spins in a diamond that can network with others via photons).
Another example: Quantum dots in III-V semiconductors (GaAs, InAs, etc.) – historically, the first two-qubit spin gate was in GaAs double quantum dots (Petta et al. 2005 did a SWAP gate). GaAs electron spins have coherence limited by nuclear spins (Ga and As nuclei are 100% abundant spin-3/2 each), causing faster decoherence (~microsecond dephasing unless refocused). Efforts to mitigate that (nuclear spin narrowing, using nucleus-free materials like graphene quantum dots, etc.) existed. But eventually people moved to Si for long coherence. Still, GaAs quantum dots are easier to fabricate and were important in prototyping gate concepts.
Hole spin qubits in Ge/Si: Already mentioned in silicon, but separate from those are Ge/SiGe quantum wells where hole mobility is high and holes have heavy-hole/light-hole states that can be used as qubits. Some results: 2021 Delft group entangled two hole spins in a germanium double quantum dot.
Topological spin qubits vs physical spin: Real anyons aren’t realized yet, but “topological qubits” (like Majorana zero modes) conceptually rely on effective spin-like degrees of freedom encoded nonlocally. That will be in the topological section. However, something like exchange-only qubits (a type of spin qubit using 3 spins, known as a Decoherence Free Subspace qubit or Loss-DiVincenzo’s encoded qubit) have been tried in GaAs. Also, singlet-triplet qubits (using two-electron spin states in a double dot) is another variant – requiring only charge measurement to read out (via whether two spins form singlet or triplet which have different energy in a magnetic field gradient). Those were more common in GaAs, but also done in Si.
Key Academic Papers
The NV center was first isolated as a single qubit in 1997 (Gruber et al.) when researchers saw optically detected magnetic resonance of a single NV. Later, in 2004, Jelezko & Wrachtrup demonstrated coherent control of a single NV electron spin at room temperature, and in 2013, the first small quantum network was shown by entangling two NV centers separated by meters via photon interference (Hanson et al., 2015 performed loophole-free Bell test with NVs). Other important papers for NVs in computing were the realization of a two-qubit conditional quantum gate in a NV-nuclear system (Jelezko et. al. 2004), blind three-qubit exact Grover search on a NV-center platform (Gustiani and DiVincenzo 2021) and error correction on a 3-qubit NV system (Taminiau group 2016). NV’s key advantage is long coherence (ms) at room temp for its electron spin, and seconds for its nuclear spin.
Quantum dot spin qubits in GaAs: Loss & DiVincenzo (1998) theoretical basis; Petta et al. 2005 first coherent oscillations of two-spin singlet-triplet. Also, GaAs had first spin qubit single-shot readout by Elzerman et al. 2004.
How It Works (NV Center)
NV center’s ground state is a spin triplet. With a strong magnetic field or small one, you isolate a two-level subspace (0 vs +1, often). You can manipulate it with microwaves or even with optical excited states (two-photon Raman). NV is special: it can be polarized into |0⟩ by shining green light (it optical pumps to m_s=0 preferentially). Readout: shine green, if it’s |0⟩ it fluoresces bright red, if |±1⟩ it is dim – enabling single-shot readout via photon counting. Two NVs can be entangled by interfering photons each NV emits (entanglement swapping). Within one NV, you can have a nearby carbon-13 nuclear spin (if present) or the host N nuclear spin to use as additional qubits (with very long memory but slower to control via RF). People have made small registers: e.g., an NV electron plus 2 or 3 nuclear spins entangled, which can implement small algorithms or error correction.
How It Works (Quantum Dot in GaAs/Others)
Similar principle as silicon: confine electron in double dot, use exchange coupling for two-qubit gates. But faster dephasing due to nuclear spins means heavy usage of echo and dynamically decoupling pulses to get decent performance. GaAs spin qubits achieved decent gate fidelities (~90% by 2010s) but not as high as Si later did, thus focus moved. However, GaAs and InAs quantum dots are used for hybrid qubits like quantum dot coupled to a photon (quantum dot molecule in microcavity can generate entangled photon pairs or act as a spin-photon interface for networking – e.g., using an InAs quantum dot as a single-photon source entangled with an electron spin state).
How It Works (Hole Qubits)
In Ge, holes have strong spin-orbit, one can use electric field to rotate spin. This means faster gates but also more sensitivity to charge noise. The trade-off is being studied. But coherence in Ge is pretty good as it can be isotopically purified too (Ge has some isotopes with no nuclear spin as well).
Comparison To Other Modalities
NV centers and similar solid-state defects are halfway between atomic and solid-state: they have fixed position in a solid but discrete energy levels like atoms, accessible at variety of conditions. NV is unique in being an optically addressable spin qubit at room temperature. That means for certain applications like sensors or small quantum repeaters, NVs excel (they’ve been used to detect magnetic fields at nanoscale, etc.). However, scaling NV centers into a large quantum computer is difficult: fabricating many NVs in specific positions, and making them talk to each other, is challenging. Entangling distant NVs via photons is possible but low success probability and rates currently.
Quantum dots in III-V vs in Si: The physics is similar, but Si has advantage of no nuclear spins (if purified) whereas GaAs always has nuclear noise (though some mitigation can be done by polarizing nuclei). Also, silicon industry pushes Si qubits. So III-V spin qubits have somewhat fallen out of favor for quantum computing, though they still are used for spin-photon interfaces (since GaAs or InAs quantum dots in optical cavities can produce photons at convenient wavelengths for fiber, etc. – something silicon can’t do because silicon is an indirect bandgap and can’t easily emit photons).
Current Development Status (NV and Defects)
NV centers: Companies like Quantum Diamond Technologies, ID Quantique (for sensors) and Bosque (Quantum Network companies) are working on NV or similar centers. The quantum computing demonstration beyond a few qubits hasn’t scaled due to difficulty of coupling many NVs. Another center, Silicon Vacancies (SiV) in diamond – can be integrated in photonic crystal cavities better, and people have entangled SiV with photons and demonstrated some basic gates. NVs are used in quantum network testbeds (e.g. a 3-node network with entanglement swapping was shown by Delft in 2021 using NVs, achieving entanglement between nodes separated by 60 km fiber). For computing, NV probably will serve as small memory nodes in a distributed scheme rather than scaled on chip.
Current Status (Quantum Dot Spins in III-V)
Largely replaced by Si for computing, but still used in photonic applications. There’s research into hybrid spin-charge qubits like “spin qubit coupled to superconducting resonator” bridging to microwave quantum bus (Yale and others did experiments coupling an InAs nanowire double quantum dot spin to a transmon qubit, achieving a high-fidelity transfer recently). Also, donors in silicon had a big success in 2022: UNSW (Simmons group) made a 10-qubit register of donor nuclear spins (all controlled via a grid of electrodes) in silicon – a major integration of donor qubits in silicon. They have thus both donors and quantum dots in silicon path.
Advantages (NV/defects)
- NV: Long coherence at room T for certain tasks, optical interfacing easily, can be used as quantum repeaters or memory in networks. Each NV-nuclear system is like a small 2-3 qubit quantum processor that is well isolated (maybe useful for specific tasks or distributed computing).
- QDs in III-V: Direct optical transitions in the same material allow spin to photon conversion. For instance, one can map an electron spin state to the polarization of an emitted photon via cavity and selection rules. This is key for quantum interconnects (like linking spin qubits with photonic channels). Also, fabrication of III-V QDs has matured for single photon sources (InAs QDs can be grown self-assembled, etc.).
Disadvantages
- NV: Hard to scale beyond a few qubits per site; positioning NVs or integrating many is tricky (though there’s work in implanting arrays of NVs at certain spacings). Also, optical readout is photon-starved (loses a lot of emitted photons, requiring sensitive detectors), and entangling operations via photons are probabilistic and slow (entangling two NVs might take milliseconds per attempt due to waiting for photons and success probability <1%). Also, each NV’s local environment (like nearby random other spins) can differ, causing inhomogeneity.
- Solid-state spin coherence issues: If host has nuclear spins (like natural diamond has 1.1% C-13 nuclear spins), they cause decoherence. One can isotopically purify carbon to 0.01% C-13 which NV experiments do to extend T2 to ~2 ms. In general, any defect qubit demands careful materials prep.
- Integration with classical control: For NV networks, needed classical logic to orchestrate photon measurements and feed-forward (like if entanglement succeeded or not). So time overhead.
Impact On Cybersecurity
NV centers or similar are not likely the ones that will break encryption because scaling to the required qubit numbers is far from their strength. They are more relevant to quantum communication than to large-scale computation. If anything, NVs help build quantum repeaters that secure communications now, rather than threatening them.
However, there is one angle: if by distributed quantum computing, a network of many small quantum processors (like NV centers with a few nuclear qubits each) could be connected via photons to act as a larger computer, one might bypass some scaling issues. It’s a bit sci-fi yet, but conceptually, e.g., 100 nodes each with 10 qubits if entangled and orchestrated could act as 1000-qubit machine. That’s extremely challenging and would be very slow (due to network latency), so not ideal for factoring large numbers which requires lots of two-qubit gates. So practically, NVs and similar are not a direct threat to crypto, but rather an enabler of quantum-secure communications.
Future Outlook (NV/Defects)
NV research continues for quantum networking. We expect demonstration of entangled networks (like connecting 4 or 5 NV nodes in a small network, achieving entanglement among them). Also NVs find roles in sensing: nano-scale NMR, detecting single molecules magnetic fields – not computing, but an important quantum tech application.
For defect qubits in general, new defects like rare earth ions in solids (e.g. Eu in YSO crystals) are studied since they have narrow optical lines and spin states – potential for quantum memory and moderate qubit count in solid.
Future Outlook (Quantum Dot & Alternative Spins)
Possibly the integration of spin qubits with photonic links: e.g., a quantum computer that uses silicon spin qubits for processing and uses an interface (like an embedded quantum dot or a coupling to an optical cavity) to send qubit states out as photons for networking or interconnect between chips. There is active work to get a photon interface for spins – if that succeeds, spin qubits become even more powerful because you can connect chips quantum mechanically (like like linking 100-qubit silicon chips with photonic buses yields a larger system).
Also, exotic proposals like molecular spin qubits (like the article from ScienceDaily above which shows interest in designing molecular systems with spins that can possibly form qubits arrays via chemistry). If chemists could self-assemble large arrays of molecular spins with controlled interactions (like metal-organic frameworks with spin centers), that might be another modality (quantum cellular automata concept in chemistry perhaps). But that’s far out and largely exploratory.
Summary for spin qubits: Spins in various platforms form a versatile category of qubits – they can be bound in semiconductors (quantum dots, donors), in crystal defects (NV, etc.), in molecules or on surfaces (like atoms on surface as IBM did for classical QCA concept), etc. The common thread is using magnetic degrees of freedom with often impressive coherence and using known techniques (ESR/NMR). The field began with NMR QCs (ensemble of spins in molecules) and has evolved to single-spin control in solid-state. Spin qubits tend to be small and potentially scalable, but coupling them at scale and controlling them uniformly is hard. Nonetheless, they remain one of the most promising qubit types especially in the context of silicon and semiconductor industry support.